The Development of a Scientific Fact
What
is a Scientific Fact? Chronology
of a Discovery Making
a Membrane
The fluid-mosaic membrane
model as a scientific fact
What the model says
The fluid mosaic model of a biological membrane defines a phospholipid
bilayer mixed with (integral) membrane proteins. The lipid bilayer,
therefore, is not a continuous layer, but contains non-lipid molecules,
in particular proteins.
Fig. Fluid mosaic
membrane model of the outer and inner membranes of a bacterial
cell wall
Abb.: LPS lipopolysaccharide;
PL phospholipid; LPP Brown's lipoprotein; X generic integral
membrane protein; EnvZ regulatory protein of the ompF - ompC
operon; F0F1-ATPase ATP synthase of electron transport chain;
IM inner membrane; OM outer membrane; PG peptidoglycan; OmpA
outer membrane protein A. (by Lukas Buehler, 1989) |
The significance of the model
The fluid mosaic model is the only accepted proper description
of a biological membrane. Its publication in 1972 by Singer and
Nicolson (Science 1972 Feb. 18 175:23 720-31) put an end to other
existing and competing models of biological membranes. These competing
models assumed triple layers, with a lipid core layer and two protein
layers, one on each side, but the proteins would not penetrate the
lipid core, i.e., did not span the membrane. The fluid mosaic model,
based on thermodynamic and functional considerations, postulated
instead that proteins as the active components of signal transduction
and transport, must and can span the entire thickness of a
lipid bilayer. Protein-lipid interaction was thought to be stabilized
through hydrophobic (fat soluble) contact points on the surface
of the proteins.
Today, the fluid-mosaic model is the only accepted model of the
structure of biological membranes, including those of animals, plants,
and microorganisms (see figure above for membranes of the cell wall
of the bacteria Escherichia coli). the use of the same structure
among all living organisms is consistent with the idea of a common
ancestor organism (or organisms) from which all modern life forms
have evolved. Since 1972 no modifications of the model have been
necessary, also individual membranes differ in molecular composition,
lipid types, or lipid to protein ratios.
To understand the stability and form of membranes one needs to
understand that they are solely determined by the aggregation behavior
of phospholipids. These lipid types in effect form extensive, planar
bimolecular films separating two aqueous compartments.
The hydrophobic core of such a thin film functions as an electrical
insulator (D=2; D stands for dielectric constant; a value of 2 represents
hydrophobic environment, a value of 80 water) allowing diffusion
of small hydrophobic solutes across membranes, but inhibits the
diffusion of charged and hydrophilic, i.e., water soluble molecules.
The incorporation of protein into these bimolecular films provides
the necessary pathways for all charged and hydrophilic molecules.
Cells have thus complete control over their membrane permeability
because they can control protein function, but not the diffusion
of small hydrophobic molecules across membranes. Cell membranes
are thus clearly specific and selective.
To understand the current model of biological membranes, one has
to know the history of the study of cell membranes, both from a
structural and functional point of view.
Early developments
The first experimental indications for a bilayer structure have
been obtained by measuring the size of a water surface that can
be covered by phospholipids extracted from red blood cells. This
area was about twice as large as the total surface area of red blood
cells used to extract lipids. It turned out this first calculation
was a lucky shot and with more accurate measurement, the two surface
ratios would not have been 2:1 due to the presence of membrane proteins.
This original finding, although not reproducible, stimulated membrane
research and guided it into the right direction.
Neurobiologist started to characterize the function of neuronal
cells by measuring action potentials which are the result of electrical
currents flowing across cell membranes (called excitable membranes
of nerve and muscle cells). Macroscopic current recordings of isolated
nerve cells in the 1940s allowed the prediction of underlying molecular
units called ion channels (the electric charges are carried by ions,
charged atoms and molecules). Although the sensitivity of electrical
recordings was not good enough at this time to measure individual
ion channels, the noise behavior of the macroscopic currents were
statistically analyzed to predict the existence of unit channels.
The macroscopic membrane currents were simply the results of the
simultaneous activity of thousands of those unit channels. These
ion channels have later been shown to be made of proteins.
It was one of the great achievements for
biophysicists in the 1960s to demonstrate conclusively that these
current recordings can be interpreted as the opening and closing
events of a single ion channel structure at a time. By the time
I got to witness this experiment, more than 20 years had passed
since the first membrane had ever been formed in vitro (black lipid
membranes; 1962 by Mueller and Rudin), and about 12 years earlier
black lipid membranes were used to study gramicidin channel activity
(D.W. Urry, 1971; M.C.Goodall, 1971).
The Montal & Mueller bilayer provided the only means to assemble
phospholipid bilayers that emulate the asymmetric distribution of
lipids found in biological membranes. These two scientists contributed
an experimental system that demonstrated for the first time that
purified membrane proteins could be reconstituted to full functionality
and recapitulate their biological function. Their contribution was
first for the nicotinic acetylcholine receptor, the voltage-gated
sodium channel, the photosynthetic reaction centers, cytochrome
oxidase, and later on the bacterial toxin channels, Bcl-2 family
of proteins, and the HIV Vpu proteins, to name a few.
The pore forming activity of gramicidin peptides had been shown
before using diverse biological membranes, such us mitochondria
and red blood cells, both depending on functional ion gradients
which are destroyed by the presence of gramicidin. Hladky and Haydon
demonstrated the unit conductance of gramicidin in 1972 which is
the functional correlate of a peptide dimer spanning a biological
(here artificial) membrane.
Fig.
Current recording from membrane containing Gramicidin D peptide
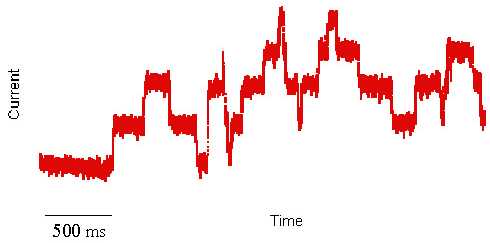 |
Click on trace to start movie. |
Rectangular transitions
up and down represent channel opening and closing respectively.
The amplitude of these transitions is uniform indicating
a unit channel activity. The number of levels thus correlate
with the numbers of active channel units within the
membrane (4 levels up from bottom level on the left).
Recording has been obtained in symmetric 500mM NaCl
and at a constant 100mV membrane potential. (Recorded by Lukas Buehler,
1997)
(QuickTime
format) of an actual multi channel recording (PCLAMP
6 software from Axon
Instruments at Molecular Devices)..
|
|
See table of key historic dates
for a more detailed outline of the development of the biological
membrane as a scientific fact. It is no longer up to the individual
experimentalist to speculate about the general structure of biological
membranes. For further reading on the general topic of scientific
facts see Ludwik Fleck.
I first heard about artificial cell membranes in the early 1980s.
I was a biochemistry student at that time and considered myself
a biologist. The very idea of using artificial systems to study
biological processes hurt my sensibility as a young student. I did
not know then that a few month later I would witness an experiment
that forever changed my mind, jump-starting a scientific career
devoted to membrane protein structure and function.
The pivotal experiment was a two-day demonstration by a graduate
student showing the mechanism of how an antibiotic compound kills
bacteria by punching holes into their cell membranes. This antibiotic,
called Gramicidin, is a peptide (small protein) able to kill bacteria
by spontaneously inserting itself into their membranes and destroying
their most important energy storing device, a proton concentration
gradient.
In plain English, Gramicidin kills bacteria by short circuiting
their battery. What was most intriguing about this experiment I
witnessed was the electrical activity that this peptide induced
when inserted into an artificial membrane. By literally creating
small ion (current) conducting channels across the otherwise electrically
insulating cell membrane, carefully placed electrodes pick up rectangular
jumps in currents exhibiting a reproducible unit conductance. Because
cell membranes are extremely thin and the channel volumes very small,
the electrical current has to be amplified about a billion fold.
This amplification demonstrated the activity of a single molecule
activity.
This is not your conventional biochemical reaction, where billions
of proteins are measured at once and the kinetic analysis of the
chemical product formation really represent the average of every
single enzyme reaction.
It is impossible from such macroscopic experiments to exactly tell
what's happening at a single active site. In the current recording
shown above (red trace), every single rectangular transition up
or down, however, does represent the activity of a single active
site, i.e., the opening or closing of a transmembrane ion channel.
This single protein activity can be observed only, if millions of
ions flow through the channel every second. The ultimate goal to
study the trajectory of a single ion through a single channel is
still beyond experimental means, but can be simulated with the help
of computers.
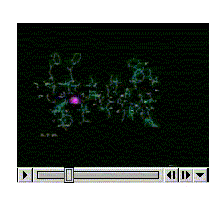 |
Molecular dynamics simulation of Gramicidin
A with Na ion.
Simulation by Robert S. Eisenberg, Rush
Medical Center, Chicago, IL; visualization by Carlos Simmerling;you
need Quicktime 3.0 or later version to view the movie.
Click on figure to start movie.
|
The amount of data accumulated over time by many laboratories has
set forth a body of evidence that allowed to call an experiment
like the one described here for Gramicidin D a single channel
recording beyond reasonable doubt. The functional characterization
of ion channels in biological membranes has proven the fluid mosaic
model correct. The model has become a scientific fact. It has become
a fact, even though nobody has ever observed the activity of Gramicidin
at this single molecule level in a real cell, a bacterial membrane.
It has become a fact even though nobody has ever seen a Gramicidin
peptide. Yet its molecular structure is known in great detail using
nuclear magnetic resonance, X-ray crystallography, or many other
spectroscopic techniques. It has become a fact because it was possible
to meticulously put together the pieces of a molecular puzzle into
a coherent system of observations. The structural and functional
characterization of biological membranes and its components is a
marvel of reductionist experimentation, of logic and deduction.
It is also the consequence of an astonishing property of biological
molecules -- to self assemble in aqueous solution.
H
o m e
Copyright © 2000-2010 Lukas
K. Buehler
|