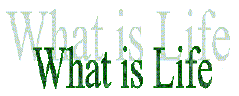
Introduction to Metabolic Biochemistry
Basic Principles of Pathway
Chemistry
Metabolism is the mechanism of living cells to extract, convert
and store energy from nutrients. Metabolism is a complex network
of chemical reactions within the confines of a cell that can be
analyzed in self-contained parts called pathways. Metabolic pathways
contribute to catabolism - the oxidative degradation of molecules,
and anabolism - the reductive synthesis of molecules. It
is important to realize that pathways, be they catabolic or anabolic
in nature, are interdependent and controlled (e.g. hormones) by
the energy needs (e.g. growth, work, digestion) and physiological
activity of an organism. The principals of metabolic pathways can
be described by four conceptually distinct categories:
Chemical
reactions |
Most
biologically important reactions are group transfer reactions,
oxidation-reduction reactions, elimination (dehydration), isomerization,
rearrangement, carbon-carbon bond chemistry |
Energy
balance |
Pathway
catalysis is determined by the activation energy, heat capacity,
substrate concentration |
Allosteric
control |
The
regulatory mechanism of pathways; pathways can be activated
and shut down by allosteric control, modulation of enzyme activity,
and enzyme availability (gene expression, translation control)
|
Cellular
integration |
Uptake,
transport, and secretion of metabolites; anabolic and catabolic
pathways are often segregated into compartments and cells spend
a lot of energy to transport substrates across biological membranes
|
Homeostasis and Thermodynamics
of Metabolic Pathways
Metabolism is a largely circular process of energy conversion
in cells of living organisms. Chemical energy is extracted from
nutrients (catabolism) and this energy is in turn used to synthesize
new molecules (anabolism) from the same type of nutrients to maintain
the structure and function of an organism. To accomplish both, say
energy extraction from and biosynthesis of proteins, metabolism
of living cells is a spatial and temporal network of chemical
reactions close to, but never at chemical equilibrium. Living
organisms maintain a state of metabolic homeostasis which
can be viewed as a steady-state throughput or flow of energy
and metabolites to sustain body functions and structures.
Nutrients provide both chemical energy and molecular building blocks
to form macromolecules like proteins, DNA, polysaccharides, and
membranes. These macromolecular structures in turn control nutrient
uptake and metabolism. Along metabolic pathways the energy content
of the initial substrate (glucose) differs significantly from the
energy content of the product (carbon dioxide and water). In thermodynamic
terms the change in Gibbs free energy, DG,
is quite large (negative value). If such a reaction would occur
at once, the chemical energy contained in sugar would be converted
to heat. Enzymes of metabolic pathways are able to capture this
energy in small portions and store it in form of internal high energy
compounds drastically reducing the amount of energy lost as heat.
Compare the caloric content of an ounce of bread to burning a small
stick of wood (wood and starch contain the same energy-rich stuff
- glucose). The heat of the flame represents the released energy.
Converting glucose to carbon dioxide and water in one step has
also one other important consequence; the reaction is irreversible
due to a prohibitively large activation energy of converting carbon
dioxide and water into sugar. Living organism have evolved metabolic
pathways that allow at least partial reversibility of the conversion
of such processes by providing many intermediate steps with small
DG values close to zero, i.e., near their
chemical equilibrium.
Note that the serial conversion of metabolic intermediates allows
the coupling of exergonic reactions with endergonic
ones. This generally allows for a reversibility of pathways. Although
some pathways are catalyzed by the same enzymes facilitating both
directions for degradation and synthesis, most enzymes operated
away from their chemical equilibrium and cells provide two separate,
non-reversible pathways - one for degradation and one for biosynthesis.
This allows for additional control by the cell to switch between
biosynthesis of new macromolecules and the energy requirements to
fuel those biosynthetic pathways, and also allows differences in
metabolism and control of metabolism from cell type to cell type,
or species to species.
Free
energy |
The relation
between the change in free energy and standard free energy
is given by:
DG
= DG°'
+ RT ln{products/substrates}
It is
important to always distinguish the free energy change (the
actual change of the reaction in vivo) from the normally
tabulated standard free energy change DG°'.
|
Standard
free energy
(not physiological) |
When
DG
= 0, the reaction is at its chemical equilibrium, we can measure
and tabulate the standard free energy change: .
DG°'
= -RTln{products/substrates}
Reaction
performed at standard temperature T = 25°C and pH = 7
(physiological pH), and adjust the starting substrate concentration
to 1M
|
Metabolic pathways follow a logic or concept that once understood,
makes it easy to analyze and explore any pathway. The first step
of a metabolic pathway normally includes the activation of the substrate
into a high energy compound (also referred to as 'committed step').
Take as an example the first step in glycolysis
(anaerobic glucose catabolism) where one molecule of glucose is
phosphorylated before it can be used for oxidative catabolism:
ATP + Glucose Û ADP + Glucose-6-P
DG°' =
-4.3kcal/mol (a) (DG
-12kcal/mol)
This reaction can be viewed as the sum of two separate reactions,
a spontaneous one (exergonic) and one that requires energy (endergonic):
ATP + H2O Û ADP + H3PO4
DG°' =
-7.5kcal/mol (b; exergonic)
Glucose + H3PO4 Û
Glucose-6-P + H2O
DG°' =
+3.2kcal.mol (c; endergonic)
The phosphorylation of glucose with inorganic phosphate costs +3.2kcal/mol
and because of this large activation energy the reaction will not
spontaneously occur in aqueous solution. ATP hydrolysis on the other
hand is an exergonic reaction yielding -7.5kcal/mol. ATP
(C00002) is indeed an unstable molecule in water and hydrolyzes
spontaneously, albeit at a slow rate. The energy of this reaction
is distributed as heat. Catalyzed by enzymes the energy of this
reaction can be harnessed to power biosynthesis; here phosphorylation
of glucose, an high energy compound.
The use of high energy
compounds in cellular metabolism
High energy compounds power metabolic pathways. By definition high
energy compounds are those with a standard free energy DG°'
of more than 6kcal/mol). The most important and widely used high
energy compound is ATP and the related nucleotides CTP,
UTP, and GTP. The major source of ATP comes from respiration
(mitochondrial process depend on molecular oxygen) and photosynthesis.
If oxygen or light are not readily available, other high energy
compounds serve as emergency fuel to restore ATP levels. These are
the so called phosphagens like phosphocreatine in vertebrates
and phosphoarginine in invertebrates. Except for ATP which serves
as a multipurpose energy donor, most high energy compounds are used
for specific metabolic pathways only (but never only one). Cytosine
triphosphate (CTP) is used in phospholipid
synthesis by generating activated intermediates (e.g. CDP-choline).
Uridine triphosphates are used for complex carbohydrate
synthesis (glycogen, cellulose) by generating activated sugars (e.g.
UDP-glucose). Guanosine triphosphate is important in protein synthesis.
Phosphocreatine is used mainly in skeletal muscle to provide chemical
energy for intense work and assure a constant supply of ATP. The
enzyme creatine kinase (EC
2.7.3.2) catalyzes the transfer of the phosphate group between
the two metabolites. Because the change in free energy of ATP regeneration
from ADP and phosphocreatine is only slightly negative, the reaction
is readily reversible and mainly dominated by the equilibrium
concentration of the reactants.
An other important activated chemical group besides phosphate ester
bonds are the acyl thioesters (acyl-CoA) of short chain compounds
(acetyl-CoA)
and long-chain fatty acids for lipid metabolism. Numerous other
high-energy intermediates are activated in order to promote group
transfer reactions. Their formation is directly or indirectly dependent
on the energy available from ATP.
The Importance of Enzymes
Glucose phosphorylation does not happen spontaneously by simply
mixing glucose with ATP in aqueous solution. The coupling of
an energy yielding reaction with an energy consuming one is done
by enzymes. Often enzymes catalyze the two reactions in series by
providing a structural scaffold that optimally orients the two reactants
(substrates) to promote a group transfer reaction from donor
X to acceptor Y. The enzyme may serve as an intermediary binding
site of the transferred group. Importantly, while the two steps
(b and c) shown above each depend on water as co-substrate,
no H2O is involved in the enzyme mediated coupled process
(reaction a).
Enzyme also provide exquisite substrate specificity. In liver,
glucose phosphorylation, the transfer of an high energy phosphate
bond from ATP to glucose is catalyzed by hexokinase 2 (E.C.
2.7.1.1 for human isoform; or glucokinase EC
2.7.1.2 for invertebrates and microorganisms).
Oxidation-Reduction Reactions
All energy yielding process are ultimately dependent upon enzymatically
catalyzed redox reactions. The most important one for energy metabolism
involve biological membranes with bound electron transport processes
like photosynthesis and oxidative phosphorylation. Biological oxidation
is the primary provider of energy for cellular anabolism, the reductive
synthesis of metabolites, by furnishing mobile hydrogens, and phosporylating
energy by combining hydrogens with oxygen to form water coupling
this process to the production of ATP in the form of oxidative phosphorylation.
Central to the oxidation-reduction processes are the vitamin
B group containing coenzymes nicotinamide-adenine dinucleotide
(NAD) and nicotinamide-adenine dinucleotide phosphate (NADP,
(C00006; oxidized form); NAD
(C00003; oxidized form; not phosphorylated at the adenosine ribosyl
C2 position). Being part of the appropriate enzymes the oxidized
nicotinamide ring of NAD+ or NADP+ extracts
a hydride (H:-) from a wide variety of simple metabolites
in a process known as dehydrogenation. The enzymes catalyzing
the reduction of nicotinamide containing coenzymes are called dehydrogenases.
In a typical reaction two hydrogen atoms (including their electrons)
are removed from the substrate to produce the oxidized form of the
donor. The fate of the two hydrogens differs: one hydrogen with
two electrons (H:-), a hydride ion, is transferred to
the nicotinamide ring to produce reduced NADH or NADPH while the
other hydrogen is released into solution as a free proton (H+).
The generic form of a redox reaction mechanism catalyzed by enzymes
with NAD as cofactor is shown.
The use of these nicotinamide based redox reactions provides versatility
and reversibility. Under most cellular conditions, the free energy
change is small and dehydrogenases catalyze both oxidative and reductive
reactions. Many different types of substrates are used as partners
for NAD and NADP - carbohydrates,
lipids, and amino acids. The coenzymes are diffusible and facilitate
the shuttling of hydrogen atoms and electrons among different dehydrogenases
that belong to different pathways. The different phosphorylation
state of NAD and NADP provides a control mechanism to use the respective
coenzymes for different classes of pathways. The phosphorylation
does not affect the redox potential of the coenzymes (see below),
but the affinity of the molecules for specific proteins. The example
of a redox reaction discussed here is the anaerobic regeneration
of NAD+ reduced during glycolysis by the reduction of
pyruvate to lactate.
NADH
+ H+ + Pyruvate Û NAD+
+ Lactate
This reaction is catalyzed by lactate dehydrogenase (human
enzyme E.C. 1.1.1.27). Lactate dehydrogenase couples the two
half-reactions:
NAD+ + 2H+ + 2e- Û
NADH + H+
Eo' = -0.32V
Pyruvate + 2H+ + 2e- Û
Lactate
Eo' = -0.19V
The standard reduction potential Eo' is used to quantify
redox reactions instead of the change of standard free energy. A
negative reduction potential indicates a reduction reaction, i.e.,
the binding of two electrons (and two concomitant H+).
The half-reaction with the more negative Eo' will act
as electron donor or reducing agent. The metabolically more important
regeneration of NAD+ is the aerobic (oxygen dependent) process
of using the reductive power of NADH for the synthesis of ATP (note
that positive Eo' values indicate spontaneous reactions).
NADH + H+ + ½O2 Û
NAD+ + H2O
Eo' = +1.14V
This process is strictly dependent on the presence of molecular
oxygen and is called oxidative phosphorylation. During the oxidation
of glucose to carbon dioxide and water, most of the reducing power
is not generated by glycolysis (in the cytoplasm of the cell), but
the tricarboxylic acid cycle, also known as Citric Acid or Krebs
cycle (in the cell's mitochondria). The complete aerobic oxidation
of one molecule of glucose yields 30-36 molecules of ATP (only 2
come from glycolysis), of which 90% is captured in the form of reducing
power and converted to ATP through oxidative phosphorylation in
the mitochondrial inner membrane. In the process, six units of carbon
dioxide and six water molecules are generated.
Glucose (C6H12O6) Û
6CO2 + 6H2O
~ 30-36 ATP
Again, the chemical energy of the covalent bond structure of glucose
is captured in small steps involving many metabolic intermediates
rendering the process partially reversible, also the overall process
is not. Humans can regenerate glucose from the metabolic intermediate
pyruvate but not CO2. Humans as we all know are not able
to synthesize glucose from 'scratch', i.e., carbon dioxide, a process
known as photosynthesis in plants and some microorganisms.
Respiration and photosynthesis are catalytically possible only
because of the coordinated activity of hundreds of proteins that
belong to deferent sets of pathways in different compartments of
cells and/or organisms. Understanding the structural and functional
complexity that provides reductive synthesis of glucose as well
as oxidative degradation is the same as understanding the mechanism
of cellular metabolism.
Pathway Control Mechanisms
Metabolic pathways can be controlled at three levels. First, the
amount of enzymes available through transcription (gene expression),
translation, and protein turnover (proteolytic degradation). Second,
the catalytic activity of an enzyme is controlled by allosteric
modulators, activators and competitive inhibitors (agonists and
antagonists, respectively), and post-translational modifications
such as phosphorylation, acetylation, and glycosylation under the
control of hormones, growth-factors, and neurotransmitters. Third,
substrate availability (concentration) is controlled by steady-state
equilibrium and compartmentalization. The latter depends on active
membrane transport systems (pumps, transporters; chemical energy
required) and the passive diffusion via substrate specific membrane
proteins (ion channels, facilitators; no chemical energy required;
diffusion is controlled by concentration gradients).
Go
back to table of contents
H
o m e
Copyright © 2000-2003
Lukas K. Buehler
|