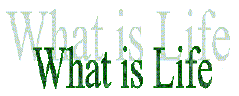
Fatty acid metabolism
Lipid diversity
(KEGG
summary pathway)
The term lipids includes a divers group of structurally
distinct hydrophobic and amphipathic molecules.
Fatty
acids |
Fatty acids are a group of negatively
charged, linear hydrocarbon chains of various length and
degrees of oxidation states. The negative charge is located
at a carboxyl end group that is completely deprotonated
at physiological pH values (pK ~ 2-3). The length of the
fatty acid 'tail' determines its water solubility (or
rather insolubility) and amphipathic characteristics.
Fatty acids are components of phospholipids and sphingolipids
which are part of biological membranes, fats (triglycerides)
which are used as energy storage devices inside cells
(adipose tissue), function as fat transport vehicle in
the blood (high and low density lipoproteins), and through
covalent attachment are used for protein modification.
Important members of this groups of fats are palmitic
acid (C16) and stearic
acid (C18) and the respective monounsaturated C18
oleic
acid(C18:1;D9).
An unsaturated (oxidized) fatty acid contains
a C=C bond in a cis conformation with the D9
indicating the unsaturated bond between C9 and C10.
|
Fats
(triglycerides) |
Fats are the mono-, di-, and triacylglycerol
forms of fatty acids. Triglycerides (triacylglycerols) are
neutrally charged, entirely hydrophobic, i.e. reduced molecules.
The mono- and diacylglycerides are metabolic intermediates
in phospholipid synthesis, while the triacylglycerol (or triglycerides)
are the fat molecules used to store chemical energy in a water
free, compact state. This latter is the main function of adipocytes
(fat storage cells) in adipose tissue of mammals. Fat storage
provides an anhydrous form (devoid of water) because of the
hydrophobic packing of fully saturated fatty acid chains.
The energy content per gram fat is 9 kcal as compared to 4kcal/g
for carbohydrates. |
Phospholipids
|
Phospholipids are diacylglycerol derivatives
with a hydrophilic, zwitter ionic, often charged headgroup
at position C3 of the glycerol backbone. Fatty acyl chains
(R groups in figure below) of most membrane phospholipids
are C16 and C18 members, with the acyl chain R2 usually unsaturated.
Phosphatidic
acid (C00416) is the biosynthetic precursor of all phospholipids
used for cell membrane synthesis and triglycerides. |
Steroids
(and steroid
hormones) |
Steroids (hormones) are signaling molecules
derived from cholesterol. They readily diffuse across biological
membranes. They form a class of hormones with target receptors
inside the cell. Examples of steroid hormones are Testosterone
and Progesterone, the male and female sex determining hormones
in higher animals. Non hormonal steroids are cholesterol and
their derivatives like ergosterol and cholic acid all of which
serve as lipid components of eukaryotic membranes. Specific
steroid membrane lipids are unique for different groups of
organisms. Cholesterol
(C00187; the parent structure of all steroids), for example,
is a membrane component found in large quantities in mammals
only, while plants are devoid of this particular lipid as
membrane component. |
Eicosanoids
|
Eicosanoids are specialized fatty acid
derivatives in cell membranes that function in processes like
inflammation. Prostaglandins
(C02064) and Leukotrienes
are the two major groups of this class of lipids. Their role
is part of the immune response of higher organisms (mammals).
|
Fat-soluble
vitamins |
Fat-soluble vitamins (vitamins A, D,
E, K) are a mixed group of linear and cyclic p
-electron systems. Vitamin D is synthesized as part of
the sterol biosynthetic pathway MAP00100.
The vitamin A group is synthesize by the retinol metabolism
|
Lipids
and their role in metabolism
About 40% of the bodies caloric intake is derived
from lipids and almost all of these calories come from fats, the
triacylglycerols.
The fatty acid composition in terms of saturation (oxidation forms)
is not uniform but varies with the origin. Plant fats contain
more polyunsaturated fatty acids and animal fats contain more
saturated fatty acids as well as cholesterol. Polyunsaturated
fats are essential for humans because animals are not able to
synthesize those on their own. Most lipids, however, have metabolic
functions contributing to membrane structures and signaling. Arachidonic
acid (C20:4) is a fatty acid which plays a central role
as precursor for prostaglandin synthesis. Phospholipids are synthesized
from diacylgycerolphosphate, a negatively charged phospholipid
precursor and signaling molecule itself, carrying various hydrophilic
and/or charged headgroups that determine the surface charge and
chemical properties of biological membrane surfaces.
Fatty
acid degradation
(KEGG
pathway MAP00071)
The use of fatty acids for energy production and inter conversion
of biosynthetic precursors is predominantly performed in liver.
The liver may play a modifying part in fat storage and retrieval.
The major source of lipids entering the liver does so in free fatty
acid form released form adipose tissue and transported in the systemic
blood plasma complexed with albumin. Fatty acid oxidation yields
twice the usable chemical energy that carbohydrates can deliver.
As an example, 130 mols of ATP result from the oxidation of one
mol of palmitic acid (C16:0), as compared to 38 mols of ATP from
one mol of glucose. On a weight basis, the caloric yield from fatty
acids is about double that from carbohydrates; 9kcal/g from fat
vs. 4kcal/g from carbohydrate or protein).
Fatty acid oxidation occurs in three major steps. In a first energy
consuming step, the fatty acid is activated by forming a high
energy thioester bond with HS-CoA. These activated fatty acid
units can be used for the synthesis of phospholipids and fats (triglycerides).
Second, the activated acyl-CoA is oxidized and C2 units removed
in the form of Acetyl-CoA in a cyclic process called beta-oxidation.
Third, acetyl-CoA moieties are funneled into the citric acid cycle
for a final round of decarboxylation-oxidation reactions. This pathway
is located in the mitochondrial matrix directly coupling the reducing
equivalents in the form of NADH + H+ to oxidative phosphorylation
and thus ATP synthesis.
Fatty acids are found in free form in the cytoplasm and need to
be transported across the inner mitochondrial membrane in a controlled
fashion. The activated fatty acid is first transferred by a mitochondrial
outer membrane protein carnitine acyl transferase I
(1) complex from acyl-CoA to acyl-carnitine. The latter is transported
across the inner membrane into the matrix compartment by carnitine
acyl translocase. There, the fatty acid is reesterified with
an CoA unit by the matrix enzyme complex carnitine acyl transferase
II (2).
Beta oxidation of fatty
acids
The process of beta oxidation is named after the carbon atom in
the beta position of the fatty acyl-CoA which becomes the most oxidized
during the cyclic redox reactions that remove C2 units in form of
acetyl-CoA from the fatty acyl chain. The beta carbon becomes the
new carboxyl end of the shortened (n-2) fatty acyl-CoA. The
oxidation steps are strictly analogous to the reaction steps in
the citric acid cycle converting succinyl-CoA to oxaloacetate involving
an initial oxidation by acyl-CoA
dehydrogenase (EC 1.3.99.3; driven by FAD reduction), an
hydration by enoyl-CoA
hydratase (EC 4.2.1.17), and a second oxidation by hydroxyacyl-CoA
dehydrogenase (EC 1.1.1.35 driven by NAD+ reduction).
A C2 unit is released by beta-ketothiolase
(EC 2.3.1.16) to produce acetyl-CoA and a shortened acyl(n-2)-CoA.
The latter is recycled until the acyl chain is shortened to its
acetyl-CoA end product and oxidized by the citric acid cycle enzymes.
The acyl-CoA dehydrogenase is specific for the length of the acyl
chain being oxidized. Three types of the dehydrogenase exist in
mitochondria; type
I (EC 1.3.99.12; long chain) which oxidizes C12-C18 fatty
acids, type
II (EC 1.3.99.3) which oxidizes C4-C14 fatty acids, and
type
III (EC 1.3.99.2; butyryl dehydrogenase) which oxidizes
C4 and C6 acyl-CoA substrates. The energy yield per cycle is 5 mols
of ATP for each round, 2 mols per FADH2 (goes into complex
II) and 3 mols per NADH/H+ (goes into complex I). The
energy balance for palmitic acid (sixteen carbon atoms) is:
CH3(CH2)14CO-S-CoA + 7H2O
+7CoA + 7FAD + 7NAD+
Þ 8CH3-CO-S-CoA + 7FADH2 + 7NADH
+ 7H+
The completion of the degradation process (coenzyme oxidation)
requires the citric acid cycle which yields an additional 96 mols
of ATP for all 8 acetyl-CoA units oxidized in the process. The total
energy yield of palmitic acid oxidation results in some 130 mols
of ATP, 34 units from the beta-oxidation cycle and 96 form the citric
acid cycle.
Beta oxidation of odd numbered fatty acids yields a (C2)
acetyl-CoA and (C3) propionyl-CoA end product during the very last
cycle. Propionyl-CoA cannot be further oxidized as such and is converted
to methyl-malonyl-CoA by propionyl
carboxylase (EC 4.1.1.41). This is an energy consuming step
using 1 mol of ATP per mol of propionyl-CoA. The net reaction is:
propionyl-CoA
+ CO2 + ATP = (D/L)-methylmalonyl-CoA
+ ADP + Pi
The methylmalonyl is formed as racemic mixture containing equal
amounts of the D- and L-enantiomer. The (D)methylmalonyl-CoA is
isomerized to succinyl-CoA by methylmalonyl CoA mutase:
(D)-methylmalonyl-CoA = succinyl-CoA
Note that this reaction is also used for the degradation of hydrocarbon
side chains of the amino acids valine, leucine, and isoleucine;
(S)-methylmalonyl is the same as L-methylmalonyl; from KEGG pathway
MAP00280)
Similarly, unsaturated fatty acids need special enzymes
to provide the beta oxidation intermediate trans-D2-enoyl-CoA, the
substrate of enoyl-CoA hydratase. Desaturation can result
in two unusual degradation intermediates: the cis-D3-
and the cis-D4-enoyl-CoA. The beta oxidation
intermediate, however is a trans-D2 isomer.
The cis-D3-enoyl-CoA intermediate is
isomerized to the trans-D2 form by isomerase
(EC 5.1.2.3). The cis-D4-enoyl-CoA form
is converted in two steps to cis-D3-enoyl-CoA
intermediate. The first step is catalyzed by acyl-CoA dehydrogenase
(the first enzyme in the beta oxidation cycle) to form 2,4-dienoyl-CoA
which in turn is isomerized by 2,4-dienoyl-CoA
reductase to cis-D3-enoyl-CoA.
Fatty
acid synthesis
(KEGG
pathway MAP00061
and MAP00062)
Apart from two polyunsaturated fatty acids (linoleic acid, C18:2;
and alpha-linolenic acid, C18:3) the human body is able to synthesize
all other fatty acids required either for structural lipids in membranes
or for storage purpose. Fatty acid synthesis and their further use
for phospholipids and triglycerides is referred to as lipogenesis.
Any metabolite that yields acetyl-CoA during its degradation is
a potential supplier for lipogenesis, the most important being carbohydrates.
In general, it can be understood that excess carbohydrates beyond
the body's energy needs will be converted into fat. Lipogenesis
is not a simple reversal of beta oxidation, but uses an entirely
different pathway for the regeneration of fatty acids from acetyl-CoA
precursors. The reductive synthesis of fatty acids is a cytoplasmic
process carried out by a multienzyme complex called the acyl
carrier protein (ACP). The acyl chain is covalently linked to
the sulfhydryl prosthetic group of ACP. The reduction-oxidation
steps require NADPH (rather than FADH2 and NADH found
during beta-oxidation).
There are three major processes involved in the reductive synthesis
of fatty acids. First, acetyl-CoA has to be transported across the
inner mitochondrial membrane into the cytoplasm. Second, the true
substrate for ACP is malonyl-CoA, a C3 acyl thioester that is formed
by the carboxylation of acetyl-CoA to malonyl-CoA. Third, the first
end product and intermediate for further lipid biosynthesis is palmitic
acid, the C16 acyl derivative. For all other lipogenetic processes,
protein complexes other than ACP are required.
Fatty acid synthesis is a cytoplasmic process. All acetyl-CoA must
be exported from the mitochondrial matrix via citrate (first step
in Krebs cycle). In the cytoplasm, citrate is split into acetyl-CoA
and oxaloacetate. The latter, if not used for gluconeogenesis, is
transported back into the mitochondrial matrix. Oxaloacetate concentration,
thus, plays a crucial role in connecting carbohydrate, lipid, and
energy metabolism. In the cytoplasm acetyl-CoA is activated by acetyl-CoA-carboxylase
(EC 6.4.1.2) to malonyl-CoA
(C00083). This C3 acyl intermediate is the immediate precursor for
fatty acid synthesis. The enzyme contains biotin as prosthetic group
catalyzing the following two reactions:
CO2 + biotin-Enz + ATP Þ carboxybiotin-Enz
+ ADP + Pi
carboxybiotin-Enz + acetyl-CoA Þ
malonyl-CoA + biotin-Enz
This reaction is analogous to that catalyzed by pyruvate carboxylase.
This committed step reaction of malonyl-CoA formation is a major
control point of fatty acid synthesis. Activated acyl units are
now transferred from CoA to a phosphopantetheine
sulfhydryl group (C01134) linked to a serine residue on ACP
for subsequent chain elongation reaction.
ACP is a subunit of a larger enzyme complex, the fatty
acid synthetase (EC 2.3.1.85). The flexibility and length (2nm)
of the phosphopantetheine unit are critical for the function of
the fatty acid synthetase complex. In yeast, the complex contains
12 subunits, with six alpha and six beta subunits. In mammals, the
complex is a dimer with 3 domains and 7 catalytic sites. Once covalently
linked to the ACP subunit, the activated substrate is not released
but moved by the pantetheine arm from catalytic site to catalytic
site through the cycle as described below. The first step uses an
acetyl-ACP and a malonyl-ACP to form the C4 acyl unit acetoacyl-CoA,
and releasing CO2. The carbon dioxide unit is stripped
off from malonyl-ACP during acyl condensation reaction. In fact,
the carbon atom released in this step is the same as the one incorporated
into malonyl-CoA. The subsequent steps in fatty acid synthesis are
the reversal of the beta oxidation steps. The keto group in the
beta position is first reduced by NADPH to form a hydroxyl group.
A dehydration step introduces a C=C which is reduced by a second
NADPH to the fully saturated hydrocarbon (C4) unit butyryl-CoA.
The synthesis cycle repeats itself with the condensation of a second
malonyl-ACP (from a second acetyl-CoA) to the C4 acyl to form a
unsaturated beta-keto-acyl-ACP. The following reduction, hydration,
reduction reactions form a fully saturated C6 acyl-ACP. This cycle
repeats until the C16 palmitoyl-ACP is formed. At this point the
fatty acid is released from the acyl carrier protein as palmitoyl-CoA.
The net reaction and energy consumption of forming one molecule
of palmitoyl-CoA from acetyl-CoA units is:
8 acetyl-CoA + 7ATP +14NADPH +14H+ Þ
palmitoyl-CoA
+ 7ADP + 7Pi + 7CoA + 14NADP+ + 7H2O
Further elongation and oxidation can occur in either the mitochondrion
or endoplasmatic reticulum. Palmitic acid (C16:0) and the C2 elongated
form stearic acid (18:0) can be unsaturated to their respective
monounsaturated forms palmitoleic acid (C16:1) and oleic acid (C18:1).
This process is catalyzed by the ER membrane and provides fatty
acids for phospholipid biosynthesis. Fatty acids (palmitic acid)
may be transported back to the mitochondrial matrix for oxidation.
Regulation of fatty acid
metabolism
Fatty acid metabolism requires a balance between degradation and
synthesis according to the energy need of cells and an organism
as a whole. It is obviously dependent on the metabolism of carbohydrates
and proteins. In humans the liver plays a role in lipid blood homeostasis
analogous to its role as glucostat organ. For the control of beta
oxidation the major regulatory mechanism is the availability of
substrate. In liver, fatty acids come from three major sources:
(i) as fatty acids released by extra cellular lipase from triglycerides
coming from fat absorption in the form of chylomicrons, (ii) as
unesterified (free) fatty acids in blood plasma (complexed with
albumin) originating from adipose tissue (adipocyte lipase), and
(iii) from intracellular triglycerides where a liver specific lipase
deesterifies triglycerides releasing free fatty acids. The cellular
lipases of adipocytes and hepatocytes are under hormonal control,
with glucagon activating the lipase and insulin inhibiting it. Other
hormones that activate lipase are epinephrine and nor-epinephrine,
and ACTH
(C02017; adrenocorticotropic hormone). These positive stimulators
all work through cAMP mediated kinase phosphorylation.
A second regulatory mechanism is metabolic control related to the
energy charge of the cell. Beta oxidation is strictly coupled to
oxidative phosphorylation and depends on the ADP/ATP ratio, with
a high ratio (low energy charge) stimulating degradation. High ATP
levels stimulates both fatty acid synthesis as well as phosphatidic
acid synthesis. ADP however accelerates beta oxidation and the oxidation
of acetyl-CoA in the citric acid cycle. The regenerated ATP will
help shift the balance back to synthesis mode.
A third control mechanism is the transport of fatty acids across
mitochondrial inner membranes. Fatty acid transport is mediated
by the carnitine acyl-transferase system. Here is a link between
carbohydrate and fatty acid metabolism. The cell avoids beta oxidation
while synthesizing fatty acids from glycolysis (high energy charge).
Malonyl -CoA functions as an inhibitor of the mitochondrial carnitine-acyltransferase
I in the mitochondrial outer membrane. If glucose is abundant (red
control function in figure), malonyl-CoA levels are high inhibiting
fatty acid transport into mitochondria and engaging acetyl-CoA for
fatty acid synthesis.
Ketone bodies
(KEGG
pathway MAP00072)
The reason for the latter control of fatty acid entry into mitochondria
lies in the fact that fatty acids, once inside the matrix compartment,
will be oxidized to acetyl-CoA which can be used for TCA oxidation,
retro-transport into the cytoplasm or, when abundant, is transformed
into acetoacetate
(C00164), the precursor of so called ketone bodies. Ketone
body excess are formed in liver when glucose levels are low and
fatty acid concentrations are up. Low pyruvate concentration reduces
the availability of oxaloacetate and thus overloads the citric acid
cycle with acetyl-CoA from both pyruvate and fatty acid degradation.
Acetyl-CoA molecules are then combined to acetoacetate and is in
turn converted to either beta-hydroxybutyrate (the reduced
form of acetoacetate) or acetone, the product of a spontaneous
decarboxylation reaction of acetoacetate in the presence of protons.
In liver, ketone bodies cannot be metabolized any further and are
secreted into the blood pool where they are catabolized in muscle
and brain cells alongside glucose. Excess ketone bodies found in
the blood are removed by the kidney in form of ketonuria, which
is an indicator of metabolic acidosis, a disorder resulting from
high levels of ketone bodies in the blood.
The heart and brain are organs that rely on ketone bodies as fuel
molecule. While skeletal muscle under strenuous activity uses glycolysis
as major energy production pathway, resting muscles primarily burn
fatty acids and ketone bodies, while neurons burn glucose and ketone
bodies. Both organs strictly depend on respiration.
The ketone body beta hydroxybutyrate is oxidized in neurons and
cardiac muscle mitochondria to produce NADH and acetoacetate. The
latter can be activated by succinyl-CoA to acetoacetyl-CoA and succinate.
Acetoacetyl-CoA is then cleaved by thiolase to two units of acetyl-CoA
for further oxidation in the citric acid cycle. The cardiac muscle
is optimized to derive most of its energy from the oxidation of
acetoacetate.
Go to table of contents
|