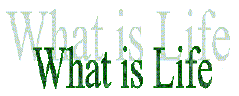
Nitrogen metabolism
Overview
It is the polymeric nitrogen containing compounds proteins and
nucleic acids that define the major attributes of organism such
as function and structure. Operation and mechanism of metabolic
pathways is provided by proteins. Genetic information is stored
in nucleic acid polymers. Each of the monomer of these macromolecules
has an individual metabolic pathway. In addition, the monomeric
nucleotides are essential for energy turnover as key intermediates
in all metabolic pathways and also as second messenger
molecules, often in form of cyclic nucleotides.
Amino acids contribute to carbohydrate synthesis via gluconeogenesis,
to fat synthesis or energy production via acetyl-CoA, and special
nitrogen compounds such as catecholamines (neurotransmitters),
thyroid hormones, creatine(-phosphate), the protoporphyrin ring
(heme), and contribute to nucleic acid and phospholipid synthesis
as nitrogen group donor.
Microbial nitrogen
fixation
(KEGG
pathway MAP00910)
All nitrogen metabolism is based on a recycling of ammonia NH3
in its neutral or charged form NH4+(ammonium
ion). Ammonia, however, is not a major form of nitrogen on earth,
instead it has to be replenished to support a growing need of
life forms. As simple as it may sound, a growing bacterial culture
needs raw material in form of small organic and inorganic molecules.
NH3 ultimately is derived from atmospheric N2.
In a process called nitrogen fixation, some bacterial species,
the symbiotic eubacteria Rhizobium (in plant root nodules)
and the archaea cyanobacteria (formerly blue-green algae)
contain an enzyme complex for the reduction of molecular nitrogen
to ammonia. This is the nitrogenase complex and contains
Fe-S and Mo-Fe cofactors for the transfer of electrons from ferredoxin
to N2. The process of nitrogen reduction is extremely
energy dependent. The triple bond energy in molecular nitrogen
is 225kcal/mol and the industrial production of ammonia requires
temperatures of 500 degrees Celsius and a pressure of 300 atmospheres.
Rhizobium uses 8 reducing equivalents and 16 ATPs:
N2 + 8e- + 8H+ + 16ATP
+ 16H2O = 2NH3 + H2 + 16ADP +
16Pi + 8H+
This reaction is catalyzed by the hetero-oligomeric protein complex
composed of a reductase and a nitrogenase part. The reductase
is a homodimer containing a 4Fe-4S cluster and an ATP binding
site at the subunit interface. The nitrogenase
(EC 1.18.6.1; PDB entry 1N2C) is a hetero-tetramer with a subunit
stoichiometry of a2b2.
The ab interface contains the so called
P cluster (containing two 4Fe-4S clusters) which oxidizes the
reductase and is oxidized by the Mo-Fe cofactor which contains
two Mo-3Fe-3S clusters comprising the N2 binding site. For a complete
structure of nitrogenase complex from Azotobacter Vinelandii
click
here.
The reductase (PDB
structure from Clostridium Pasterianum; EC 1.19.6.1) contains
an 4Fe-4S complex used to oxidize ferredoxin, which is supplied
either by photosynthetic membranes (PSI) or from oxidative catabolism. The
reductase donates 8 electrons in succession to the nitrogenase
cofactor, a molybdenum-iron containing active center, where one
molecule of N2 is reduced in the presence of protons
to 2 NH3, and H2 as a byproduct. The reduction
catalysis is powered by sixteen ATP molecules hydrolyzed by the
reductase subunit. Molecular oxygen is a strong inhibitor of the
nitrogenase Mo-Fe cofactor and is removed by the plant oxygen
binding protein leghemoglobin in the root nodules.
Dietary nitrogen
The majority of useful nitrogen for animal metabolism comes from
proteins in the form of reusable ammonia (NH3). Nitrogen is fixated
in form of ammonia by microorganisms (see chapter on amino acid
synthesis) and all 'higher' forms of life (eukaryotes) depend
on this primordial source of nitrogen extracted from the air.
The 'usefulness' of proteins depends on four distinct properties:
1. total amount of protein ingested
2. digestibility of proteins
3. amino acid composition of proteins
4. total caloric intake
Digestibility and amino acid composition define the biological value
of dietary proteins. Hair and skin keratin is non-digestible and
useless as such. Pretreatment like heating can improve protein usefulness.
Animal proteins are a better mix for our diet than plant proteins
based on their amino acid composition.
Nucleic acids, on the other hand, are not needed as dietary supplement.
Excess nucleic acid in the diet is degraded and secreted and most
nucleic acid synthesis in cells is provided by protein degradation
(in form of amino acid precursors). Since all nitrogen containing
compounds are dependent on protein supply, protein deficiency is
one of the major nutritional problems in the world. This is specifically
important for children and pregnant women, since the developing
organism has a several fold higher need for proteins in the diet
than the adult. Low protein intake results in lowered protein synthesis
and thus in a lower supply of essential proteins involved in food
digestion (proteases) and blood plasma transport (lipoproteins).
Essential amino acids
Some amino
acids may be synthesized in human cells, some however cannot.
The latter are referred to as essential amino acids meaning that
they are required dietary components. The non-essential amino
acids, however, can be interconverted into each other or synthesized
de novo from carbohydrate, nucleic acid, or lipid intermediates,
provided that an adequate source of total nitrogen is available.
For essential amino acids there is no metabolic pathway for de
novo synthesis except in bacteria and plants. Humans therefore
need a daily balanced intake of those essential amino acids. Meat
and milk provide such a balanced amino acid diet by virtue of
the evolutionary relationship with between animals and humans.
The list of the essential amino acids for human protein synthesis
includes the branched amino acids Isoleucine, Leucine,
and Valine, the sulfur containing Methionine, the
hydrophilic amino acids Lysine and Threonine, and
the aromatic amino acids Phenylalanine and Tryptophan.
The amino acids Arginine and Histidine are synthesized
in human cells, but only slowly and thus can be considered essential,
if they become rate limiting factors for protein synthesis.
Nitrogen balance
The nitrogen balance is an indication of protein synthesis and
degradation. A positive nitrogen balance indicates that
the intake of nitrogen containing compound exceeds the nitrogen
lost from the body. A positive nitrogen balance correlates with
a net synthesis of proteins and nucleic acids. This obviously
describes a state of growth of an organism - childhood,
pregnancy, recovering from illness. The opposite results in a
net degradation of proteins. Less nitrogen is taken up than is
lost, is a state of negative nitrogen balance. The omission
of essential amino acids, and this needs to affect only one type,
results in a negative nitrogen balance, since it will be rate
limiting for protein synthesis. A healthy body is characterized
by a nitrogen equilibrium (steady state equilibrium) where intake
and loss of nitrogen are equal. Here is a quote from Murray's
"Essentials of human metabolism":
" In a way this equilibrium description satisfies our
common sense observation of non growing adults. The interpretation
would be that the body uses only as much protein nitrogen from
the diet as necessary to replace digestive enzymes, gastrointestinal
cells (GI) lost in the feces, or any degenerated tissue components,
such as skin cells or erythrocytes that wear out during normal
use. ... Why then does the body have such high requirements specifically
for protein as well as for essential amino acids? Why could these
energy requirements not be met almost entirely by increased carbohydrate
and lipid intakes? The answer to these questions requires a different
concept of metabolism than is implied by the terms 'chemical equilibrium'
or the replacement of components due to wear and tear."
The answer lies in protein turn over, continuous exchanges of material
and energy with our surroundings. The body requires energy to transport
metabolites, needs to make sure the proteins are in good shape and
that the structures inside and outside of cells are not compromised
by hazardous materials. The entire human body is in a true steady-state,
a flow of components recycled through rounds of synthesis and degradation.
The non-growing body is essentially constantly renewing itself creating
stability and the illusion of non activity, while in fact its components
are being continuously exchanged. Turn-over rates of proteins are
measured in minutes, hours, or days depending on the protein and
its cellular location. Not only can the organism replace damaged
enzymes, it can also quickly adjust the levels and types of proteins
according to metabolic needs. Indeed, extracellular transport mechanisms
(lipoproteins) are coupled to intracellular protein synthesis and
degradation pathways.
The key regulatory element of this turn-over process is the nitrogen
balance reflected as the free amino acid pool. This pool is regenerated
by dietary proteins and tissue protein degradation and is the
source of protein synthesis as well as nitrogen secretion while
maintaining nitrogen level homeostasis.
Turnover rates are best described as biological half-life time.
An estimated 2 to 3 weeks has been given for a complete turnover
of all body proteins (with a considerable variation). The turnover
rate of individual proteins or specific families of proteins may
be less than an hour. In actual numbers the rate of protein synthesis
every day is estimated at about 500g or nearly five times the
average dietary intake. There is obviously a highly efficient
amino acid recycling machinery at work. This, in short, is the
significance of amino acid metabolism.
Liver nitrogen metabolism
The liver is the main metabolic organ utilizing amino acids for
tissue protein synthesis, heme formation, pyrimidine and purine
synthesis (nucleotide precursors), ketone body and carbohydrate
formation, de novo synthesis of non-essential amino acids,
and finally excrete surplus nitrogen via the urea cycle. The liver
thus is the gatekeeper of the nitrogen balance in animals, its
intake and excretion. Because of its central role of regulating
and coordinating body metabolism, protein turnover in liver is
particularly fast. This ensures a reliable supply of (intact)
blood plasma proteins, and liver resident proteins obviously affect
liver metabolism which affects all other tissues, too. Finally,
some proteins may be rapidly degraded to provide a constant level
of free amino acids for the formation of ketone bodies, carbohydrates,
nucleic acids, and heme. Hormonal control (glucocorticoids) makes
sure that a starving body first breaks down proteins from non-essential
organs like skeletal muscle, while liver enzymes for gluconeogenesis
and urea cycle (nitrogen decontamination of the body) are enhanced.
The liver acts as an aminostat. Free amino acid levels
in blood plasma as well as plasma proteins are maintained at constant
levels despite fluctuations in intake and tissue demand.
Glutamate
(C00025) and glutamine
(C00064) are the two important amino acids in recycling ammonia
in our body instead of excreting it as waste in form of urea
(C00086). Glutamine is synthesized from glutamate by incorporation
of an NH3 into the carboxyl group forming an amide. This step
requires ATP and is catalyzed by glutamine
synthetase (EC 6.3.1.2). The coupling of glutamine synthesis
with ATP hydrolysis renders the reaction irreversible. The back
reaction - the regeneration of glutamate from
glutamine - is catalyzed by glutaminase
(EC 3.5.1.2), which deaminates glutamine via a hydrolysis reaction.
The concerted control of these two enzymes is responsible for
the maintenance of the glutamine pool in blood. An example of
controlling the NH3 levels is enhanced gluconeogenesis in specialized
organs such as muscle and brain. Carbohydrates are synthesized
from amino acid sources increasing the cellular ammonia levels.
They are secreted by the peripheral tissues in form of glutamine
(to avoid that nitrogen is excreted from the body) which is taken
up by hepatocytes where the NH3 is re-used for amino acid and
nucleotide synthesis.
Aminotransferases
Aminotransferases are a class of enzymes responsible to attach
and remove amino groups from alpha-carbons of amino acids
and keto acids. Aminotransferases (or transaminases) link
amino acid metabolism with other pathways, most importantly the
citric acid cycle. The reaction catalyzes the transfer from
an alpha amino acid to an alpha keto acid. The transferase using
alpha-ketoglutarate and alpha-glutamate as acceptor and donor
group, respectively, takes a central role in the linkage between
amino acid metabolism and citric acid cycle. This reaction is
coupled with the enzyme glutamate dehydrogenase which catalyzes
the amination-deamination equilibrium between alpha-ketoglutarate
and glutamate. Thus, the interplay of the two enzymes glutamate
transaminase (EC 2.6.1.1; transferase) and glutamate
dehydrogenase (EC 1.4.1.2) is essential in the control
of nitrogen balance in the body.
Aminotransferase reactions involve little change in free energy
(they catalyze the reaction close at its chemical equilibrium)
and the direction of the catalysis is essentially controlled by
the concentration levels of the reactants. The dehydrogenase activity
is controlled by the redox potential of the cell in form of NADH.
The amination (NH3) reaction is coupled to a reduction step using
NADH/H+ (oxidized) and alpha-ketoglutarate (reduced) while producing
glutamate, NAD+ and water.
Aminotransferase reactions depend on vitamin B6, namely its derivative
pyridoxal-phosphate
(C00018), which acts as a coenzyme in the reaction, temporarily
binding the transferred amino group. The pyridoxal phosphate group
converts to pyridoxamine phosphate during the catalysis. Pyridoxal
phosphate, however, is quite a versatile coenzyme being used in
enzymes catalyzing the following reactions by temporarily accepting
the transferred reactant (hint: click on the link above to pyridoxal
phosphate and explore the long list of enzymes (117) that use
this functional group):
- transamination
- decarboxylation
- deamination
- racemization
- aldole cleavage
- elimination and replacement reactions at b
carbons and g carbons
Glutamate is the major partner for many amino acids during aminotransferase
activity. Among those amino acids are aspartate (aspartate-glutamate
aminotransferase), tyrosine (tyrosine-glutamate aminotransferase),
and alanine (alanine-glutamate
aminotransferase EC 2.6.1.2). The respective keto acids are
oxaloacetate (aspartate), pyruvate (alanine), and hydroxyphenylpyruvate
(tyrosine). The central step in glutamate/alpha-ketogluatarate amination
and transamination can be illustrated by the amination of pyruvate
to alanine. The net reaction
pyruvate + NH3 + NADH + H+ Û
alanine + NAD+
is catalyzed in two steps. First by glutamate dehydrogenase:
alpha-ketoglutarate + NH3 + NADH + H+ Û
glutamate + NAD+
followed by alanine-glutamate aminotransferase:
pyruvate + glutamate Û alanine
+ alpha-ketoglutarate
All steps are reversible. The dehydrogenase reaction occurs in
the mitochondrial matrix where it directly interacts with NAD+
and alpha-ketoglutarate. The dehydrogenase is under allosteric
control of the energy charge of the cell. High levels of ATP and
GTP inactivate the enzyme while high levels of ADP and GDP activate
it.
Non-essential amino
acids
All non-essential amino acids except for tyrosine and cysteine
are derived and are dependent on transamination from glutamate.
Proline, ornithine, arginine obtain their carbon units
and amino nitrogen from glutamate. Alanine, serine, glycine
obtain their C3 carbon units from glycolytic intermediates and
the amino nitrogen from glutamate (Note: glycine is a C2 amino
acid derived from serine by decarboxylation; see one carbon metabolism).
Aspartate derives its carbon backbone from oxaloacetate
(C4) and amino nitrogen from glutamate. In fact, glutamate-dehydrogenase
in combination with any aminotransferase is capable of forming
any non-essential amino acid, given the occurrence of the proper
alpha-keto acid and a source for ammonia. This process is called
reductive amination (see above formation of alanine from
pyruvate). The main purpose of reductive amination is to recycle
NH3 instead of excreting it in form of urea and to preserve other
amino acids which could serve as amino group donor.
Reductive amination is the first of three processes in liver
for ammonia incorporation. The second important process is the
formation of glutamine, which serves as a reservoir for ammonia
for all organs and is maintained as blood glutamine levels by
liver cells. Glutamine serves as transport mechanism of NH3 between
organs. Third, liver can form carbamoyl-phosphate, which is necessary
for the formation of pyrimidine bases of nucleotides and the production
of urea via the enzymes of the urea cycle.
Urea cycle
(KEGG
pathway MAP00220)
The urea cycle is a liver resident process removing nitrogen
in form of ammonia to be excreted from the body. The cycle involves
two amino acids which are not used for protein synthesis. These
are ornithine and citrulline. Ornithine has a role
analogous to that of oxaloacetate in the citric acid cycle. It
provides the carbon backbone and works as a catalytic carrier,
but is not itself used up in the cyclic reaction.
Ornithine has a terminal amino group that serves as a hook or
handle for the incoming carbamoyl
phosphate (C00169), a small molecule formed from CO2
and NH3 and ATP as phosphate donor. Carbamoyl-phosphate is catalyzed
by carbamoyl-phosphate
synthetase (EC 6.3.4.16; forming carbamoyl- phosphate)
in the mitochondrial matrix and requires the hydrolysis of 2 molecules
of ATP. Carbamoyl is transferred to the ornithine amino group
driven by the hydrolysis of its phosphate ester bond. Citrulline
(C00327) is the product of this reaction and will be transported,
together with aspartate, out of the mitochondria and into the
cytoplasm. There, aspartate and citrulline are combined into the
metastable intermediate argininosuccinic
acid (C03406) using one molecule of ATP as energy source.
Argininosuccinate is cleaved into arginine and fumarate. The latter
is recycled back into the mitochondria for use by the citric acid
cycle, while most of the arginine is converted by arginase
(EC 3.5.3.1) to urea
(C00086) and ornithine, thus completing the cycle. Like ornithine-carbamoyl
transferase, arginase is a liver specific enzyme (in the cytoplasm)
and only in those animals (mammals) which convert their nitrogen
waste to urea. Note that in muscle most arginine synthesized is
instead used for protein synthesis and creatine
(C00300) formation. The phosphorylated creatine-P is used as an
intermediate energy storage device under anaerobic conditions
in skeletal muscle.
Fumarate is used to regenerate aspartate used up by urea formation.
This is done by funneling fumarate back into the citric acid cycle
and removing it in form of oxaloacetate (alpha keto acid) which
can equilibrate with aspartate (alpha amino acid) catalyzed by
an amino transferase reaction. Both the Krebs and urea cycle are
thus strictly interrelated with the former providing essential
intermediates plus carbon dioxide and energy in form of ATP (from
oxidative phosphorylation, strictly speaking).
The urea cycle is part of two cellular compartments, the mitochondrial
matrix which performs the biosynthetic part of the precursors
citrulline and aspartate, and the cytoplasm, which after formation
of arginino-succinate cleaves this intermediate into three different
products, one of which is the net product (urea), the other two
(ornithine and fumarate) are recycled into the matrix compartment
and their respective cycle to start a new round of urea formation
Ammonium ion metabolism
Nitrogen can also be excreted as ammonium. This process is controlled
by the kidney and is used to control the blood plasma pH. The
blood plasma pH, however, is determined by other factors as well,
such as organic acids (amino acids) and carbonic acid (CO2
levels). Ammonium metabolism in kidney functions to depose H+
in urine. In a first reaction, kidney enzymes deaminate glutamine
in two steps to a-ketoglutarate. The
first side chain deamination is catalyzed as simple hydrolysis
and is not reversible.
This process is stimulated by inorganic phosphate. The free ammonia
equilibrates with protons to ammonium:
NH3 + H+ = NH4+
and is trapped in the charged form inside kidney cells. The non-electrolytic
ammonia is freely diffusible across cell membranes. Glutamine
is the nontoxic form of NH3 and shuttles it between liver and
kidney in the blood plasma. The kidney functions as H+ sink and
protons are disposed in form of NH4+ while
maintaining charge homeostasis using phosphate or acetoacetate.
Go to table of contents
|