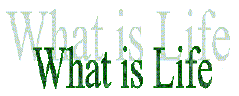
Photosynthesis
Overview
(KEGG
Pathway MAP00195)
All energy consumed by biological systems ultimately comes from
light energy trapped in reduced carbon skeletons by the process
of photosynthesis.
H2O + CO2 Þ
(CH2O) + O2
This simple net reaction is catalyzed by a very complex enzyme
machinery in plants and photosynthetic bacteria. Photosynthesis
traps light to convert photon energy into chemical energy in a process
reminiscent of oxidative phosphorylation. This membrane bound process
is referred to as light reaction and depends on an electron transfer
chain powered by reducing equivalents extracted from water. The
chemical energy produced - ATP and NADPH - is used for for the synthesis
of carbohydrates by carbon fixation (Calvin cycle or dark
reaction).
Chloroplasts
This chapter presents the plant photosynthetic apparatus.
It is located in specialized organelles called chloroplasts
with a complex membrane system that allows the separation of charges
to convert electromagnetic energy (photons) into electrochemical
energy (proton gradient) and eventually into chemical energy in
the form of ATP (phosphoryltransfer potential) and NADPH (reducing
power) and ultimately as carbohydrates, fats and amino acids..
Photosynthetic membranes
and the light reaction
The light reaction is a membrane bound process. The photosynthetic
membranes in plants contain two photosystems, photosystems I and
II, or PS I and PS II. Photosystem I captures the energy
of single photons by excitation of electrons in magnesium ions in
green pigments called chlorophyll (chlorophyll
a, C05306; see pathway map details below). The color of the
chlorophylls indicates the absorption maxima of the system, i.e.,
the ability to capture light energy of photons at a specific wavelength.
Photosystem I is coupled to an NADP+ - reducing enzyme
to produce reducing power in the form of NADPH/H+. This
reduction requires a steady supply of electrons and protons (reducing
equivalents) These reducing equivalents are provided by a second
photosystem which oxidizes water to molecular oxygen. Photosystem
II uses the energy from a second photon to reduce plastoquinone
QH2. The reduced quinone are deoxidized by a cytochrome
bf complex separating electrons from protons generating a proton
gradient. The proton gradient is coupled to H+-ATPases
similar to those in mitochondria synthesizing ATP from ADP + Pi.
Photosynthesis is not simply a reversal of oxidative degradation
of carbohydrates and oxidative phosphorylation in mitochondria.
The difference lies primarily in the source of high potential
electrons. For oxidative phosphorylation the electrons come
from hydrocarbon and carbohydrate structures, whereas in photosynthesis,
the electrons have to be energized in the magnesium center of chlorophylls.
The trick is to find a primary source of electrons to replenish
the electrons extracted from chlorophylls by means of electromagnetic
excitation. This primary source is H2O. The energy potential
of electrons in water is very low (water is not a reactive solvent)
and it is a special metal ion complex (contains manganese) in PSII
that has an even lower energy potential for electrons oxidizing
water into the radical O. and hydrid H-, two
very unstable, reactive intermediates. Photosystems II and I are
coupled by the cytochrom bf complex and the electrons extracted
from water by PS II (strong oxidant) and upon photon activation
is transported by the electron transport chain via plastoquinone
(weak reductant) to the cytochrome bf complex and from there to
PS I via plastocyanin (weak oxidant) where it is activated by a
photon into activated PS I (strong reductant) which in turn
is capable of reducing NADP+. The photosystems simply
provide a means of increasing the high reduction potential necessary,
using two photon activated steps, to push electrons from water to
NADP+.
Photosystem
II |
Photosystem II is a multi subunit complex (10 subunits) with
a molecular weight > 600kDa. It catalyzes the light driven
electron transfer from H2O to plastoquinone (Q;
oxidized
form C02061). The net reaction for one photon (hn)
is:
2Q + 2H2O Þ O2
+ 2QH2
Plastoquinone exists in three different redox states; the
fully oxidized Q (non charged), the half reduced/oxidized
semiquinone form QH.-, and the fully reduced
plastoquinol form QH2. The photosystem II protein
complex contains three subcomplexes, the light harvesting
complex II, the reaction center RC, and the oxygen
evolving unit. The light harvesting complex II (LHC2)
contains accessory pigments to capture photons. There are
seven chlorophyll a, six chlorophyll b, and 2 carotinoid units
in LHC2. The difference in the different pigments is their
maximum of absorption, which broadens the spectrum of available
sunlight to excite electrons. At the core of the complex lies
the reaction center (RC) which is a dimer consisting of subunits
D1 and D2, a special pair chlorophyll a (with an absorption
maxima at 680nm; P680), and an electron transport chain across
the thylakoid membrane consisting of pheophytin (with
2H+ instead of a Mg+ in the porphyrin
ring) and two quinones, quinone A and quinone B (plastoquinone
Q). Quinone A is tightly bound to the reaction center complex,
whereas QB is able to dissociated in the fully
reduced form QBH2 (Plastoquinol-1;
reduced QB; C02185). The electron, activated
by a photon in the special pair at the lumenal side of the
thylakoid membrane, is now located at the stromal side. The
result is a charge separation across the thylakoid membrane
which is now used by the membrane soluble plastoquinole which
diffuses across the membrane back to the lumenal side where
it is being oxidized by the cytochrome bf complex. It donates
its electrons to the cytochroms and the protons to the thylakoid
lumen aqueous compartment. Thus a proton gradient is established
in this first half reaction between PS II and cytochrome bf
complex. The oxygen evolving complex catalyzed the
oxidation of water by a manganese complex as shown. Four electrons
are sequentially extracted from 2 molecules of water producing
molecular oxygen (O2) and 4 H+ that
are used for the reduction of plastoquinone. The Mn-complex
is in its S(0) state, the fully reduced state and can donate
electrons to P680 in the reaction center. The S(4) state is
the fully oxidized state capable of extracting electrons from
water molecules.
The extraction process needs two water molecules bound simultaneously
to form one molecule of molecular oxygen to prevent the formation
and release of free oxygen radicals that would damage the
plant cell.
|
Cytochrome
bf complex |
The cytochrom bf complex essentially couples photosystem
II and I and also provides the means of proton gradient formation
by using cytochrom groups as redox centers in the electron
transport chain thereby separating the electron/hydrogen equivalent
into its electron and proton components. The electrons are
transferred to photosystem I via plastocyanin and the
protons are released into the thylakoid lumen of the chloroplast.
The net reaction of the cytochrome bf complex is:
QH2 + 2PC(Cu2+) Þ
Q + 2PC(Cu+) + 2H+
The protein complex contains 4 subunits, a 33kDa cytochrom
f, 23kDa cytochrome b563 (two hemes), a 20kDa Fe-S
protein, and a 17kDa protein.
|
Photosystem
I |
Photosystem I is a membrane protein complex with 13 subunits
and a molecular weight of > 800kDa. It catalyzes the net reaction:
PC(Cu+) + ferredoxinox Þ
PC(Cu2+) + ferredoxinred
Again, the membrane protein complex promotes a charge separation
transferring an electron from the lumenal to the stromal side
of the thylakoid membrane. The energy for the transfer comes
from light absorption by pigment P700, a special pair of chlorophyll
a, and the subsequent electron transfer via quinones A0
and A1 (vitamin
K1; reduced form C03313), a 4Fe-4S complex, and from there
to ferredoxin. The enzyme ferredoxing-NADP+-reductase,
a flavoprotein containing protein, reduces NADP+
to NADPH. Photosystem I can run in two modes. First, it promotes
the reduction of NADP+ to provide reductive power
for biosynthetic purposes. Second, photosystem I can switch
to a cyclic operation where cytochrom bf complex
serves as the electron acceptor instead of ferredoxin-NADP-reductase.
The electron cycles from ferredoxin (Fd) to plastocyanin
(PC) and back coupling to a proton transport for maximal ATP
synthesis. The cyclic mode is stimulated when NADPH levels
are high. P700 is the PS I special pair chlorophyll a which
is photon activated (P700*) to drive the cycle.
|
Carbon
fixation (dark
reaction) - Calvin Cycle
The dark reaction of photosynthesis catalyzes the fixation of carbon
dioxide into carbohydrates. This process is performed by the Calvin
cycle which resembles the reversal of the pentose phosphate pathway.
The fixation step is catalyzed by the enzyme RUBISCO (ribulose1,5biphosphate
carboxylase/oxygenase):
Ribulose1,5biphosphate
+ CO2 Þ 2 Glycerate-3-phosphate
The synthesis of D-Ribulose 1,5-bisphosphate (C01182) can be found
in KEGG pathway maps MAP00630 (Glyoxylate and dicarboxylate metabolism)
and MAP00710 (Carbon fixation). The carboxylase/oxygenase
enzymes catalyzing this carbon dioxide fixing reaction have E.C.
numbers 2.7.1.19 (R) and 4.1.1.39 (R), respectively. Glycerate-3P
is phosphorylated and reduced to glyceraldehyde-3-phosphate, a glycolysis/gluconeogenesis
intermediate and, in a combination of transketolase and transaldolase
reactions analogous to the pentose phosphate pathway, recycled to
provide ribulose-5-phosphate. The latter can be further phosphorylated
by ATP hydrolysis to form ribulose-1,5-biphosphate. The net energy
balance of six rounds of the Calvin cycle to produce one mol of
hexose is thus:
6CO2 + 18ATP
+12NADPH + 12H2O Þ C6H1206
+ 18ADP + 18Pi + 12NADP+ + 6H+
The Calvin cycle's hexose product is fructose-6-phosphate which
can be converted to glucose-6-phosphate by the gluconeogenetic pathway.
Glucose-6-phosphate can be used for starch and cellulose synthesis
(see section on glycogen metabolism).
The Structure of Rubisco is known at high resolution and
can be found at the protein data base (PDB entry for Rubisco L subunit
(biological unit is an octamer) is 1A7J.
Rubisco is a large enzyme complex on the stromal surface of the
thylakoid membrane with 8 large subunits of 55kDa each (L subunits)
and 8 small subunits of 13kDa each (S subunits). The L subunits
contain both the catalytic and regulatory sites of the complex.
The protein is activated by carbamate formation by RuBP carboxylase
activase. A CO2 unit is covalently linked to the
epsilon amino group (e -NH2)
of a lysine residue (lysine-COO-; carbamate) of an L
subunit.
Control of Calvin cycle
Carbon dioxide fixation (dark reaction) occurs during the day time
and is strictly dependent on the light reaction, i.e., the formation
of reductive power as NADPH. It does not occur literally in the
dark (at night). The metabolic activity at night instead is mainly
shifted to carbohydrate oxidation, when plants consume oxygen rather
than producing it. The control of the Calvin cycle therefore means
the control of regulation between light and dark reaction. The enzymes
of the Calvin cycle are sensitive to the proton concentration of
the chloroplast stromal compartment. The optimum pH for Rubisco
activity lies around pH 8. The proton pump driven during light absorption
moves protons from the stroma to the lumenal side to the thylakoid
membrane increasing the stromal pH. This activates Rubisco and thus
the Calvin cycle. The proton level control is coupled to magnesium
interaction (chelation) with carbamates. Magnesium stabilizes carbamate
formation and thus activates Rubisco. The key enzymes fructose
biphosphatase and sedoheptulose biphosphatase of the
Calvin cycle are also under pH control. In addition, they are sensitive
to the redox potential of the chloroplast stromal compartment. Biphosphatases
are active in their reduced state only sensing the oxidation state
of photosystem I. The mediator molecule is the small protein thioredoxin.
Thioredoxin is coupled to ferredoxin by ferredoxin-thioredoxin reductase.
Thioredoxin also inhibits phosphofructokinase. In plant cells,
light stimulates the Calvin cycle enzymes (PSI being the strong
reductant) and inhibits glycolysis.
Photorespiration
Rubisco catalyzes a second reaction that also uses ribulose1,5biphosphate
(RuBP) as substrate in the presence of molecular oxygen instead
of CO2. This process is known as photorespiration
and yields phosphoglycolate (a C2 compound) and 3-phosphoglycerate
(a Calvin cycle intermediate). Phosphoglycolate cannot be used by
the Calvin cycle enzymes and two units are converted into 3-phosphoglycerate
via a complex pathway via glyoxylate (in peroxyxysomes). Glyoxylate
is transaminated to glycine. Two glycine molecules are combined
to form serine (minus one carbon dioxide) which is oxidized to 3-phosophglycerate.
Thus, photorespiration consumes molecular oxygen (in chloroplast)
and generates carbon dioxide (in mitochondrion). Because
this process is the result of the competition of molecular oxygen
and carbon dioxide for Rubisco, photorespiration reduces the overall
yield of photosynthesis (light plus dark reaction).
C4 plants
Not all plants show the same efficiency in photosynthetic carbon
fixation. Certain plants produce high levels of CO2 which
helps reducing the energy wasting effect of photorespiration by
competing with molecular oxygen for Rubisco. These so called C4
plants have a malate/pyruvate shuttle mechanism between mesophyl
cells and bundle sheath cells to provide high carbon dioxide concentration
in the latter. Mesophyl cells carboxylate phosphoenolpyruvate to
oxaloacetate (C4), which is reduced to malate. Malate in turn is
transported into the neighboring bundle sheath cells where it is
decarboxylated to pyruvate. The released CO2 serves as substrate
for Rubisco. Pyruvate is transported back into mesophyll cells and
regenerated to phosphoenolpyruvate (see gluconeogenesis).
Go to table of contents
|