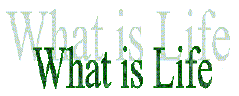
Membrane Transport
Overview
Metabolic pathways are separated not only by virtue of different
enzyme systems that catalyze their forth and back reactions (anabolism
and catabolism), but also by multiple compartmentalization. Compartments
represent aqueous solutions like the cytoplasm, extracellular fluid,
the mitochondrial matrix, or the endoplasmatic reticulum lumen all
separated from each other by membranes or phospholipid bilayers.
Weakly hydrophobic molecules (e.g. molecular oxygen O2,
carbon dioxide CO2, steroid hormones, thyroxin) rapidly
diffuse across these membranes by free diffusion. Water H2O
has a slow rate of diffusion and its flow is facilitated by the
presence of aquaporins (water channels).
This is a typical situation for polar and charged molecules. Membrane
proteins are the 'enzymes' which facilitate the transport of metabolic
intermediates between cell compartments as well as into and out
of the cell. All transport systems pertaining to metabolites can
be understood as mediated transport promoting either facilitated
diffusion or active transport. Protein mediated diffusion differs
from non-mediated diffusion of O2 and CO2
in four distinct ways:
1. the speed and specificity of the transport
2. the transport capacity is finite, i.e., exhibits saturation kinetics
3. transport can be competitively inhibited (antagonists)
4. transport can be chemically inactivated (heat, high salt)
A well studied example is the glucose transport across red
blood cell membranes (erythrocyte membrane). Comparing the diffusion
coefficient D for glucose across synthetic phospholipid membranes
(vesicle membrane; bilayer) with that of erythrocyte cell membranes
shows a 10+6 fold increase of glucose diffusion across
the cell membranes (D(bilayer) 2.4x10-10mM*cm/s; D(erythrocyte)=2x10-4mM*cm/s).
Transporter or ion channel?
Although chemical potentials (gradients) determine the direction
of diffusion across membranes (from a compartment with high concentration
to a compartment with low concentration, i.e. the [electro-] chemical
potential gradient in the case of ions), thermodynamics cannot tell
us how fast the diffusion proceeds. The difference in transport
rate between ion channels and transporters is at least 10,000 fold.
Transporters other than channels are called carriers and are subdivided
into passive (facilitators) and active (pumps) systems. Carriers
are also referred to as permeases, antiporters and symporters and
translocases. The low flux rate associated with carriers is the
prize the membrane transport system pays in exchange for substrate
specificity. Facilitated transport is tightly coupled to the chemical
potential of metabolites or a group of metabolites (representing
a pathway). They are also coupled to the energy charge of the cell
in the case of pumps. Active transport is either coupled to ATP
hydrolysis or ion gradients. The latter uses a mechanism
called flux coupling where the energy requiring transport
of the substrate (against its gradient) is coupled to the exergonic
flux of an ion (Na+; K+; H+) or in exchange for an other metabolite.
These systems work either as symporters (co-directional flux) or
antiporters (counter-flow).
Facilitated
glucose transport in humans
Glucose transporters in humans come in five subtypes called GLUT1
(gene name SLC2A1; solute carrier family 2), through GLUT5.
They are located in the plasma membrane of cells and have glucose
binding sites outside and inside the membrane. The relative affinities
of these two binding sites may differ. Different cells express different
glucose transport genes.
GLUT1
and 3 |
These two types are found in all cells (except
liver, intestinal epithelia and pancreatic beta cells) and are
responsible for a basic glucose uptake. The Michaelis-Menten
constant Km = 1mM and is slightly lower than the lower range
of healthy blood glucose concentration of 4 - 8mM. Thus, glucose
is efficiently absorbed by cells even during fasting and starving
conditions. Liver, kidney and intestinal cells can synthesize
glucose and release it into the blood circulation, a process
known as gluconeogenesis (see GLUT2). |
GLUT2: |
A low affinity glucose transporter that is typically expressed
in liver, intestinal epithelia and beta cells (insulin secretion)
of the pancreas. Its Km = 15-20mM is several fold higher than
average blood glucose levels of 4 - 8mM glucose. As result
glucose entry into liver cells (and beta cells) is normally
slow, but proportional to the glucose level in blood, while
GLUT1/3 systems are saturated and promote a steady glucose
influx into cells (e.g. in neurons). In the small intestine,
glucose absorption into mucosal epithelial cells occurs via
a Na/glucose symporter. Glucose is pumped into the cells using
the energy of the Na gradient (flux coupling) where both substrates
accumulate. Glucose subsequently diffuses via GLUT2 into the
extracellular fluid reaching the portal vein blood circulation.
Intracellular Na+ is kept low by the Na-K-ATPase on the same
basal membrane as GLUT2. The Na/glucose transporter is the
primary uptake system of intestinal glucose and galactose
during fasting conditions, when sugar levels are low. During
and after a meal, GLUT2 is also found in the apical membrane
supporting the efficient glucose uptake from the intestinal
lumen.
|
GLUT4 |
is found in adipocytes and skeletal muscle cells
and has an affinity of Km = 5mM, right at blood serum levels
of glucose (4-8mM). This receptor is upregulated by insulin.
High glucose levels, which will saturate the muscle transporter
(but not the liver/pancreas type), cause the secretion of insulin.
Insulin activates the glut4 gene and more transporter
proteins are synthesized and incorporated into the muscle cell
membrane, increasing the capacity for glucose transport in this
system (increase of Jmax; see below). |
GLUT5:
|
has a preference for fructose, the monosaccharide
found in fruit sugar together with glucose. GLUT5 is found in
the apical membrane of intestinal enterocytes allowing the diffusion
driven absorption of fructose into the enterocytes. Fructose
is thought to leave the enterocytes on the basolateral side
via the GLUT2 transporter or may be metabolically converted
to glucose before leaving the enterocytes. |
Why are there differences in the effective glucose transport mediated
by distinct GLUT transporters? Differences can be assessed (quantified)
based on the dose-response analysis where the uptake rate is measured
at increasing glucose concentration (measured as flux JB
of substrate B; Jmax indicates saturation and and constant
Km is defined as substrate concentration where
Jmax is half maximal). GLUT1&3 are saturated at
physiological blood glucose levels while GLUT2 in liver still slows
down the glucose equilibration because of its much lower binding
affinity for the substrate. The binding curve for GLUT2 at physiological
conditions is essentially linear. This simply means that glucose
uptake rates in liver are directly proportional to blood glucose
levels, exactly what one expects from a glucostat. Muscle glucose
uptake is not saturated at physiological (resting) conditions, but
will be saturated after a diet high in carbohydrates. To ensure
maximal glucose absorption by muscle cells, insulin triggers GLUT4
protein biosynthesis (gene expression regulation) effectively increasing
the transport capacity of muscle plasma membranes to over 200% normal.
Mitochondrial
carriers and shuttles
The inner mitochondrial membrane is an important selective barrier
that controls the coupling of the central respiratory energy producing
pathways - citric acid cycle and oxidative phosphorylation
- with carbohydrate, fat, and amino acid metabolism. Several
transport system have been identified that couple the citric acid
cycle intermediates with the cytoplasm, transfer reducing equivalent
from cytoplasmic NADH to matrix NADH (or FADH2), and
couple the energy state of a cell, i.e., its ADP/ATP ratio with
the electron transport chain. During glycolysis NADH is produced
and its reducing power utilized by the electron transport process
in the mitochondrial inner membrane. The nicotinamide-adenosine
dinucleotide molecule cannot cross neither the outer nor the inner
mitochondrial membrane in order to bind to complex I (binding site
on matrix side of membrane). Mammalian systems have two shuttle
systems that extract the reducing equivalent from cytoplasmic NADH
and funnel it into oxidative phosphorylation.
The first system is the glycerol-P-shuttle where dihydroxyacetonephosphate
(DHAP) is reduced by NADH/H+ to glycerole-3-phosphate
(C00093) and NAD+ by Glycerol-3-phosphate
dehydrogenase (EC 1.1.1.8). The small, reduced and negatively
charged C3 compound readily diffuses across the outer membrane porin
channels (VDAC; voltage dependent anion channel). It binds to a
dehydrogenase complex where is delivers its reducing equivalent
to an FAD coenzyme to form FADH2 and DHAP. The reduced
FADH2 donates the electron/hydrogen pair to ubiquinone
much like complex II, although this system is different from complex
II. The glycerol-P shuttle thus transports NADH equivalents against
an NADH gradient. Because the process is equivalent to the matrix
compartment succinate dehydrogenase complex (complex II) using FAD
as coenzyme, the resulting proton pumping (via quinone pool) is
only sufficient to produce two mols of ATP for every mol of cytoplasmic
NADH/H+.
The second system is slightly more complex, its intermediates tightly
coupled to the levels of citric acid cycle intermediates, but is
able to transport the full reducing equivalent of cytoplasmic NADH/H+
into the mitochondrial matrix. This system is known as malate-aspartate
shuttle. Here NADH is oxidized using oxaloacetate reduction
to malate. Malate diffuses across the outer mitochondrial membrane
(using porin). From the intermembrane space it is transported into
the matrix via the malate-alpha-ketogluatarate antiporter
in the inner membrane (for every malate entering the matrix compartment
one molecule of alpha-ketoglutarate is expelled). Malate is subsequently
reoxidized to oxaloacetate reducing NAD+ to NADH/H+.
The full reducing equivalent of cytoplasmic NADH is thus transporter
into the mitochondria.
NADH/H+ interacts with complex I (to reduce ubiquinone
QB) and three mols of ATP are synthesized for every mol
of malate transported into the matrix. Oxaloacetate cannot cross
the inner membrane because their is no transport system (carrier)
for it. Instead, oxaloacetate is converted to aspartate by the oxaloacetate-aspartat
transferase ( a transamination reaction; see nitrogen metabolism).
Aspartate is transported out of the mitochondria in exchange for
glutamate where the aspartate is converted back to its keto acid
form oxaloacetate by a cytoplasmic oxaloacetate-aspartate transferase.
The alpha-ketoglutarate and glutamate undergo an analogous transamination
reaction in both the cytoplasm and mitochondrial matrix, thus closing
the malate-aspartate shuttle system and providing a net transport
of NADH form the cytoplasm to the mitochondrial matrix.
This shuttle system is fully reversible, unlike the glycerol-P
shuttle, where the membrane bound glycerol dehydrogenase (FAD coenzyme)
cannot catalyze the DHAP reduction to glycerol-3-phosphate (for
thermodynamic reasons; see reduction potentials). The transport
system is not coupled to an ATPase or ion gradient, however, the
substrate availability determines if NADH/H+ reducing
equivalents are imported or exported from the mitochondrial matrix.
Under aerobic conditions, the citric acid cycle is fully active
producing a steady-state throughput of NADH into complex
I keeping its concentration in the matrix low and NAD+
high. As a result, cytoplasmic NADH reducing equivalent is imported
providing the necessary oxidized NAD+ coenzyme for glycolysis.
If the energy state of the cell is high (ATP accumulates) the electron
transport chain is suppressed and accumulating NADH/H+
reducing equivalents are exported from rather than imported into
the matrix and both citric acid cycle and glycolysis are allosterically
inhibited.
This malate-aspartate shuttle also links amino acid metabolism
with the energy charge and citric acid cycle of the cell. It depends
on the presence of aminotransferases. High levels of citric acid
cycle intermediates increase the capacity of the malate-aspartate
shuttle with high transfer rates of cytoplasmic reducing equivalent
into the mitochondrial matrix. Note that the carbon carriers of
the reducing equivalent are not used up in this reversible, cyclic
carrier mechanism. The malate-aspartate shuttle couples the energy
state of the cell in terms of reducing power to the metabolic rate
of the mitochondria (ATP synthesis).
ATP-ADP translocase
A third system that uses membrane bound proteins to 'read' the
metabolite concentrations is the ATP-ADP translocase in the inner
mitochondrial membrane. It is an antiporter that exchanges one molecule
of ADP for every molecule of ATP moving into the mitochondria effectively
coupling cytoplasmic ADP/ATP ratios with mitochondrial ADP/ATP ratios.
In contrast to the malate-aKG and aspartate-glutamate
antiporters, the ATP-ADP translocase is an electrogenic antiporter
because the 1:1 exchange of a dinucleotide for a trinucleotide produces
a charge difference. ATP is usually exported into the cytoplasm
and ADP imported for further ATP synthesis. ATP carries 4 negative
charges while ADP carries only 3. The result is a net export of
one negative charge. This charge imbalance is coupled to the proton
gradient, which in the first place makes the inner mitochondrial
membrane outside positive and the matrix side negatively
charged. In effect, the membrane potential component of
the proton gradient is the energy source to drive the ATP-ADP translocase
to secrete ATP4- from the mitochondrial matrix while
importing ADP3-. Despite similar binding affinities
of the two nucleotides for the translocase, ATP experiences a stronger
driving force than ADP resulting in a 30 fold higher ATP efflux
than ADP. One might expect that inorganic phosphate equilibrates
this charge imbalance because it is a necessary substrate for ATP
synthesis. Phosphate uptake into the mitochondrial matrix, however,
is mediated by a H+/Pi symporter. This transporter
carries inorganic phosphate along protons in an electroneutral manner
pushing the negatively charged phosphate against the electrochemical
gradient.
Go to table of contents
|