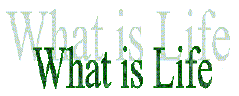
Amino acid metabolism
Amino acid metabolism
(KEGG pathway MAP01150
common amino acids for protein biosynthesis)
(KEGG pathway MAP01160
non common amino acids)
Amino acid metabolism is complex because of the large number of metabolites
involved. Amino acid metabolism can be split into those 20
amino acids used for protein biosynthesis. They also function
as precursors for the synthesis of many signaling molecules (see neurotransmitter
and nitric oxide chapter). They are distinct from the unusual
amino acids (e.g. ornithine) used for a large variety of intermediary
pathways and activated one-carbon units used for the synthesis
of aromatic, nitrogen containing compounds such as nucleic acids and
all its cofactor derivatives like nicotinamides and coenzyme A, ubiquinone,
heme and chlorophyll.
Degradation
Because of the multitude of pathways, only a selected few are presented
here demonstrating basic principles found in all other metabolic
pathways of nitrogenous compounds. Amino acids serve as precursors
for lipids, carbohydrates, and nucleic acids including ribonucleotides
used as cosubstrates and coenzymes in the production of energy (ATP,
NAD, FAD, CoA). Following the metabolic fate of carbon atoms of
dietary amino acids, they can be traced to all major metabolic intermediates
because of the close interaction of amino acid metabolism with both
the citric acid cycle and glycolysis/gluconeogenesis. These intermediates
containing carbons from dietary amino acids include pyruvate, acetyl-CoA
and acetoacetyl-CoA (ketone bodies), and the citric acid cycle intermediates
a-ketoglutarate, succinyl-CoA (heme synthesis),
fumarate, malate, and oxaloacetate.
Amino acid metabolism is 'separated' into pathways according to
the different length of carbon structures involved. These are referred
to as the C3, C4, and C5 families of amino acids. which produce
common end products during catabolism. The C3 family includes alanine,
serine (glycine), and cysteine, all of which are degraded to pyruvate.
They are glucogenic amino acids because they can directly be utilized
by the liver gluconeogensis (except their amino groups which are
excreted as urea). The C4 family of amino acids includes aspartate
and asparagine, which are degraded to oxaloacetate and are closely
linked to glutamate and alpha-ketoglutarate interconversion by amino
transferases. The C4 amino acid threonine has a separate pathway
leading to pyruvate and is a glucogenic amino acid. The C5 family
of amino acids includes glutamine, proline, arginine, and histidine,
all of which are converted ultimately to glutamate, which is deaminated
to alpha-ketoglutarate.
The non-polar C4 amino acids methionine, isoleucine and valine
are precursors for the synthesis of odd numbered fatty acids
via the intermediate propionyl-CoA (a C3 acyl-CoA). Propionyl-CoA
can be reused for succinyl-CoA (C4) synthesis (carboxylation) which
in turn serves as heme precursor. The non polar amino acid leucine,
however, undergoes a more complex degradation pathway, including
a decarboxylation-carboxylation detour leading to the formation
of acetyl-CoA and acetoacetyl-CoA (a ketone body).
Metabolism of aromatic
amino acids
Aromatic amino acids include phenylalanine, tyrosine and tryptophan.
Phe and Tyr are closely related. They contain a benzene ring which
is hydroxylated in tyrosine. Tyrosine is synthesized directly from
the essential amino acid phenylalanine. Tryptophan contains a conjugated
indole ring and its metabolism is linked to that of vitamin B (niacin
C00253). These metabolic relations give rise to an intricate nutritional
dependence. For example, a high level of dietary tyrosine relieves
the need for essential phenylalanine. Also, metabolic disorders
like the impairment of synthesizing tyrosine from phenylalanine
makes the former an essential amino acid. This lack of amino acid
biosynthetic pathways in humans is the cause of many diseases associated
to malnutrition. Pellagra is a vitamin deficiency syndrome caused
by an inadequate supply of niacin (vitamin B) because of problems
in the pathway leading from tryptophan to niacin synthesis. Vitamin
C (C00072), which is a necessary coenzyme in tyrosine metabolism,
or vitamin
B6 (C00250), which is required for tryptophan metabolism, cause
deficiencies in the metabolism of aromatic amino acids.
Degradation of aromatic ring structures is mostly performed in
liver, also many specialized cells can use the benzene and indole
rings for the synthesis of more complex, biologically important
molecules such as heme, pigments, and hormones.
Phenylalanine
(KEGG pathway
MAP00360)
Phenylalanine is first converted to tyrosine by the addition of
a hydroxyl unit. This reaction is catalyzed by phenylalanine
hydroxylase (EC 1.14.16.1). This is a liver specific enzyme
and belongs to the group of monooxygenases. The reaction requires
molecular oxygen, NADPH, and the coenzyme tetra-hydrobiopterine
(C00272). Note that the hydroxylation of the benzene ring is used
to destabilize it preparing the ring structure for breakup. The
cofactor hydrobiopterine
is oxidized during tyrosine formation. It is converted back
to its reduced form by dihydrobiopterin
reductase (EC 1.6.99.7). The reaction of phenylalanine
hydroxylase itself is irreversible.
Tryosine
(KEGG
pathway MAP00350)
Tyrosine degradation is a liver resident process. It starts out
with the transfer of its amino group to alpha-ketoglutarate by tyrosine-glutamate
aminotransferase (EC 2.6.1.5). This enzyme is specific for
both tyrosine and phenylalanine. Other L-tyrosine accepting transaminases
are less substrate specific, including aspartate
transaminase (EC 2.6.1.1) which acts on aspartate, tyrosine,
phenylalanine, and tryptophan. This latter enzyme isoform is obtained
from aromatic-amino-acid
transaminase (EC 2.6.1.57) by controlled proteolysis. The
degradation intermediate of this transaminase reaction is 4-hydroxy-phenylpyruvate
(C01179) which in turn is oxidized in the presence of vitamin C
to homogentisic
acid (C00544). This reaction is catalyzed by 4-Hydroxyphenylpyruvate
dioxygenase (EC 1.13.11.27). The ring structure of homogentisate
is subsequently broken and the linear C8 unit degraded in two reaction
steps to fumarate and acetoacetate, one citric acid cycle intermediate
and one ketone body.
Tyrosine transferase (EC 2.6.1.5) is under hormonal control.
Glucocorticoids
stimulate amino acid degradation in liver which yields fumarate
and acetoacetate. Fumarate is converted to oxaloacetate which stimulates
gluconeogenesis. Thus liver can synthesize and export glucose into
the blood plasma. Acetoacetate is secreted and used by neurons and
muscle tissue for oxidative degradation. In enteric bacteria
like E.coli, the major degradation product of tyrosine (phenylalanine)
is succinate. Also phenol, pyruvate, and free NH3 can
be derived by the action of tyrosine-phenol-lyase.
Tryptophan
(KEGG pathway
MAP00380)
Tryptophan degradation in human also yields precursors for glucose
synthesis. The first step, catalyzed by tryptophan
oxygenase (EC 1.13.11.1), is controlled by cortisol
(a glucocorticoid). Tryptophan stimulates its own degradation by
allosterically activating tryptophan oxygenase. This is an
example of a feed-forward mechanism. The product of the oxygenase
reaction is L-Formylkynurenine
(C02700), which is further degraded by kynurenine
formidase (EC 3.5.1.9). The products are formate and kynurenine
(C00328). The latter is degraded to 3-hydroxyanthranilate
(C00632) and alanine. This step requires the presence of the cofactor
vitamin B6 (pyridoxal). Hydroxyanthranilate can further be decarboxylated
to form acetoacetate. Most of hydroxyanthranilate (95%) is used
for ketone body formation. The remaining 5 percent go into pyrimidine
synthesis (nucleic acid synthesis). Tryptophan is a minor source
for the synthesis of NAD (nicotinamide dinucleotide). Most of the
nicotinamide is derived from niacin. A daily supply of NAD(P) can
be obtained from ~1mg of niacin, while it takes 60mg of the amino
acid tryptophan to accomplish the same.
Synthesis
Amino acid synthesis includes the fixation of nitrogen in form
of ammonia and the assimilation of the latter into keto acids to
form amino acids by means of glutamate dehydrogenase and
glutamine synthetase. The keto acids are provided by glycolysis
and citric acid cycle. In total, there are six anabolic pathways
for amino acids referred to as biosynthetic families. Many
non essential amino acids can directly be obtained by transamination
from glutamate to the respective keto acid (e.g. pyruvate to alanine;
oxaloacetate to aspartate). Essential amino acids have to be obtained
from dietary proteins. Plants and micororganisms have all pathways
for the net synthesis of amino acids needed for protein biosynthesis.
The synthesis of aromatic ring containing amino acids is discussed
below.
Essential
amino acids |
Non-essential
amino acids |
Histidine |
Alanine |
Isoleucine |
Arginine |
Leucine |
Aspartate |
Lysine |
Asparagine |
Methionine |
Cysteine |
Phenylalanine |
Glutamate |
Threonine |
Glutamine |
Tryptophan |
Glycine |
Valine |
Proline |
|
Serine |
|
Tyrosine |
Synthesis aromatic amino
acids in E.coli
(KEGG
pathway MAP00400)
Only microorganisms and plants have the capacity to synthesize
aromatic amino acids. Starting from intermediates of glycolysis,
the pentose phosphate pathway and/or photosynthesis (calvin cycle),
these organisms form the intermediate shikimate
(C00493) in several steps. First, shikimate is converted in three
steps to chorismate
(C00251), which serves as the committed precursor for the synthesis
of all three aromatic amino acids, but also for ubiquinone and folate
synthesis. The enzyme chorismate
mutase (EC 5.4.99.5) catalyzes an intramolecular transferase
reaction (isomerization) to form prephenate
(C00254), a precursor of tyrosine and phenylalanine. Alternately,
chorismate is converted to anthranilate in the presence of glutamine
by anthranilate
synthase (systematic name: chorismate pyruvate lyase; EC
4.1.3.27). This reaction commits chorismate towards the synthesis
of thryptophan.
Regulation
Amino acid biosynthesis is under allosteric feed back regulation.
In general, the end product of a pathway, the amino acid, inhibits
the enzyme catalyzing the first (or committed step) of its own biosynthetic
pathway. This ensures the energy saving synthesis of building blocks
for protein biosynthesis. Accumulating amino acids thus shuts down
their biosynthetic activity. A simple feed back mechanism is found
in E.coli. Here, isoleucine, which is derived from threonine,
inhibits threonine
deaminase (EC 4.2.1.16), the committed step in isoleucine
biosynthesis which forms alpha-ketobutyrate or oxo-butanoate.
For the biosynthetic pathways of more complex and branched aromatic
amino acids, however, an analogous sequential feedback mechanism
has been elucidated, as found in the bacteria Bacillus subtilis.
Simply put, the endpoint of each branch inhibits the first enzymatic
step of the immediately preceding branching point. Thus, the aromatic
amino acids do not only inhibit their early common pathways
leading to shikimate, chorismate, or prephenate intermediates. This
assures flexibility for the cell by adjusting the levels of aromatic
amino acids according to actual needs. Protein synthesis may need
different amounts for phenylalanine than tryptophan. Thus tryptophan
may shut down its own biosynthetic branch by inhibiting anthranilate
synthase, leaving chorismate mutase unaffected. Chorismate will
still be used for phenylalanine or tyrosine formation before it
accumulates to shut down the entire pathway.
Although E.coli uses the same pathways for the synthesis
of aromatic amino acids, it uses a different control mechanism to
ensure relative independence between the formation of phenylalanine,
tyrosine, and tryptophan. Instead of using this simple sequential
feedback mechanism after branching points, E.coli relies on enzyme
multiplicity meaning that E.coli uses three different
enzymes (2-Dehydro-3-deoxyphosphoheptonate aldolase) for
the early synthesis of shikimate. Each enzyme is under allosteric
control of its 'own' amino acid end product. Thus the level of enzymes
in the cytoplasm determine the level of shikimate and chorismate.
For phenylalanine and tyrosine, an additional enzyme multiplicity
control is used to allosterically suppress two isoforms of chorismate
mutase, one of which is specific for Phe, while the other binds
only Tyr. Overall, enzyme multiplicity, too, shows a sequential
feedback mechanism because of the multiple branching points of the
pathway.
Metabolism of sulfur
containing amino acids
(KEGG pathway MAP00271
for methionine)
(KEGG pathway MAP00272 for
cysteine)
Two sulfur containing amino acids are used in proteins
- methionine and cysteine. Methionine is an essential
amino acids for humans. It contributes to the hydrophobicity
of a protein. Its sulfur is non reactive. Cysteine in contrast
has a highly reactive sulfhydril group which is the cause for disulfide
bridges (cysteine pairs) in extracellular proteins (cysteine
pairs don't form in the cytoplasm, which has a reducing environment).
Cysteines can be part of catalytic sites of proteins or serve as
metal ion binding sites, e.g. for Zn coordination in the DNA binding
protein domains called Zn-fingers.
The metabolism of both sulfur containing amino acids is closely
related. Methionine is a precursor for cysteine formation in human
liver. Methionine, although it cannot be regenerated from cysteine,
can be recycled through methylation of homocysteine
(C00155) using a single carbon (methyl-) donor such as betaine (from
choline), folate, or vitamin
B12 (C05776; Cobalamin (III)) Homocysteine methylation
is catalyzed by 5-methyltetrahydrofolate-homocysteine
methyltransferase (EC 2.1.1.13) tapping into the one
carbon pool metabolism of folate and tetrahydrofolates.
Alternately, methionine is regenerated by betaine-homocysteine-S-methyltransferase
(EC 2.1.1.5). The methyl donor betaine
(C00719) is a derivative of choline. Choline is directly obtained
from phosphatidylcholine by the hydrolytic activity of phospholipase
C (PLC; EC 3.1.4.3). This reaction pathway is linked to glycine
and serine metabolism because the dimethylglycine product is reverted
to glycine.
The direct recovery of methionine in liver is part of the activated
methyl cycle transferring activated methyl groups from a THF
donor to S-adenosylmethionine
(C00019). This major source for methyl compounds, in form of activated
S-adenosylmethionine, comes from dietary methionine. Methionine
is linked with ATP to form S-adenosylmethionine releasing both inorganic
phosphate and pyrophosphate. This reaction is catalyzed by the enzyme
methionine
adenosyltransferase EC 2.5.1.6.
ATP + L-Methionine + H2O = Orthophosphate + Pyrophosphate + S-Adenosyl-L-methionine
The phosphoester hydrolysis fuels the reaction forming an activated
methyl group that can be donated to diverse acceptor molecules such
as nor-epinephrine (see Neurotransmitters) and ethanolamine (see
Phospholipids) to form epinephrine
(adrenaline) and choline, respectively. The methyl group is transferred
mostly to amino or hydroxyl groups on the acceptor. The amino acid
homocysteine cannot be used for protein synthesis and has to be
shortened by one carbon unit to cysteine in a process known as trans-sulfuration.
In this reaction, serine serves as acceptor. The short lived intermediate
cystathionine
(C02291; homocysteine -S-serine) is split in a hydrolysis reaction
to homoserine and cysteine. Both synthetase
(4.2.1.22) and hydrolyse
(EC 4.4.1.1) are vitamin B6 (pyridoxal) dependent. The reactions
are irreversible.
In E.coli cysteine
is synthesized from serine in two steps. First, serine is acetylated
to acetyl-serine by serine
acetyltransferase (EC 2.3.1.30). Acetylserine is converted
to L-cysteine by the addition of a sulfhydril group. The enzyme
cysteine
synthase A (O-acetylserine sulfhydrylase A; EC 4.2.99.8)
catalyzes this reaction.
Glycine
and tetrahydrofolate metabolism
(KEGG pathway MAP00790
for folate)
(KEGG pathway MAP00670
for one-carbon pool)
(KEGG pathway MAP00260
for glycine, serine, threonine)
Tetrahydrofolate
(THF, C00101) as discussed above is an important carrier of activated
one-carbon units. While S-adenosylmethionine only transfers methyl
groups, THF carries a great variety of activated one-carbon units
of different oxidation states:
most
reduced |
-CH3 |
methyl |
|
-CH2 |
methylene |
|
-CH=O |
formyl |
|
-CH=NH |
formimino |
most
oxidized |
-CH= |
methylene |
Carboxylation (-COOH) cannot be mediated by THF, instead always
requires biotin as coenzyme. One-carbon units are interconvertibel
(can be reduced or oxidized; see one
carbon pool metabolism) and are activated on THF on the nitrogens
N5 or N10 or both (methylene).
The major one-carbon acceptor molecules are found in the biosynthetic
pathways of methionine, glycine, purines, and deoxythymine. The
example for glycine is shown below. Glycine synthase catalyzes
a reversible reaction and thus produces either glycine or one carbon
units and ammonium.
Glycine can be regenerated from serine. As the example shows, serine
can donate a methylene (-CH2-) unit in form of an activated N5,N10-methylene-THF,
which in turn can be used to synthesize glycine from CO2 and
NH4. More glycine can be used up to generate more N5,N10-methylene-THF.
This is a de novo synthesis of one carbon units from carbohydrates.
Go to table of contents
|