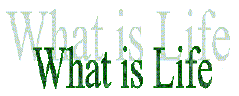
Glycolysis*
and Gluconeogenesis**
Carbohydrate Metabolism
Select the human MAP
for starch and sucrose metabolism with a colored map
of human enzymes marked as green shaded boxes.
Carbohydrates are found as monosaccharides, disaccharides, and
polysaccharides or complex carbohydrates. They function in energy
storage (starch&glycogen), signaling (glycoproteins and glycolipids,
e.g. blood group determinants), fuel the nervous system and muscle
(and virtually all cells, although there are distinct cell type
specific differences in choice of primary fuel molecule), are parts
of nucleic acids (genes, mRNA, tRNA, ribosomes), and as cell surface
markers and signaling in glycolipids and glycoproteins, are part
of connective tissue (heteropolymers; glycosaminoglycans), cell
wall components (cellulose, hemi-cellulose) made of polymers that
are enzymatically inert for most mammals to digest (except ruminants
that harbor a special digestive tract bacterium with the appropriate
cellulase enzyme). The alpha glycosidic bonds found in glycogen
and starch are metabolically available to humans.
Starch is the major source of dietary glucose. The enzymes responsible
for starch degradation are called amylases.
Other sources of glucose are sucrose,
a disaccharide glucose-fructose from fruits, and lactose,
a glucose-galactose
disaccharide from milk. Only monosaccharide species like
glucose, fructose and galactose can be absorbed via active membrane
transport systems. Special intestinal glucosidases split the disaccharides
into their monosaccharide components. Maltose is hydrolyzed by isomaltase
(oligo-1,6-glucosidase, E.C. 3.2.1.10) and, with lower efficacy,
by sucrase
(sucrose alpha-glucosidase, E.C. 3.2.1.48). Lactose intolerance
comes from a lack of lactase
in many adults, causing an accumulation of milk sugar with consequences
such as dehydration.
There is no specific carbohydrate requirement for humans except
for Vitamin
C, the C6 compound ascorbic acid. Man, some primates,
and guinea pigs lack one of the enzymes required for the synthesis
of L-ascorbic acid. This has been understood as an inborn metabolic
disorder arising during primate evolution. Consequently, L-ascorbic
acid has to be taken up with our food. All other monosaccharides
can be synthesized and are not required dietary supplements.
Once the intestinal mucosal cell of the small intestine have transported
the monosaccharides into the blood circulatory system they can pass
directly into the liver, where fructose and galactose are converted
into glucose. The principal role of the liver is to act as a blood
glucostat. Excess glucose will be stored as glycogen mainly
in liver and muscle cells or in form of metabolized fat in adipocytes.
Only glycogen, but not fats, can later be metabolized when food
intake is restricted to maintain an adequate level of glucose
in the blood stream. Fat, however, can be used for the oxidative
regeneration of ATP and reductive power (NADH).
Glycolysis
(KEGG
Pathway MAP00100)
Glucose metabolism is conserved throughout evolution, but species
and tissue specific variations are well known and of physiological
importance. The well studied metabolic pathways of glucose oxidation
for energy usage are derived from biochemical studies of mammalian
liver, muscle, and brain tissue as well as E.coli.
The major pathways are glycolysis and the pentose-phosphate
pathway. producing pyruvate
Pathway |
Substrate |
Product |
Energy
stored as |
Glycolysis |
Glucose
(1 mol) |
Pyruvate
(2 mol) |
ATP
& NADH (2 mol) |
Pentose
Phosphate Cycle |
Glucose
(1 mol) |
CO2
(6 mol) |
NADPH
(12 mol) |
Glycolysis is used by both aerobic and anaerobic organisms. Glycolysis
in human
and bacteria
are almost identical with respect to the enzymes employed, but differ
by their uptake mechanism of glucose into the cell and the end product
under anaerobic conditions. In humans glucose enters the cytoplasm
through glucose facilitators (passive
diffusion). In enteric bacteria glucose
intake is fueled by concomitant phosphorylation, while the hexose
is transported across the membrane.
Step |
Energy
yield |
1.
Committed step of glucose phosphorylation to Glucose-6-phosphate
|
-
1 ATP |
2.
Converting glucose-6-P into the ketose form fructose-6-phosphate |
|
3.
Phosphorylating fructose-6-phosphate to Fructose-1,6-
bisphosphate |
-
1 ATP |
4.
Fructose-1,6-biphosphate is split into two chemically different
trioses, glycerone-P
(or dihydroxyacetone-P) and glyceraldehyde-3-P
(GAP) |
|
5.
Glyceron-P (DHAP) is isomerized to GAP resulting in two metabolically
equivalent glyceraldehyde-3-P |
|
6.
Glyceraldehyd-3-P oxidized and phosphorylated to glycerate-1,3-biphosphate
|
+
2 NADH |
7.
Glycrate-1,3-biphosphate converted to 3-phosphoglycerate
|
+
2 ATP |
8.
Phosphate at position C3 will now be moved to position C2 forming
glycerate-2-P
|
|
9.
Elimination reaction (-H2O) producing phosphoenolpyruvate
|
|
10.
Phosphate will be transferred to form ATP and Pyruvate
|
+
2 ATP |
11.
Pyruvate to lactate reduction (under anaerobic conditions;
provides NAD+ for step 6) |
-
2 NADH |
Note: At step 7 glycolysis has regained the phosphoryltransfer
potential it invested in the first three steps to commit glucose
to oxidative degradation. The net energy gained so far in form of
ATP is zero, but the pathway yielded 2 mols of NADH. Up to here
glucose phosphorylation served as catalyst to extract reducing power.
Considering the intermediate of two mols of NADH, the net production
for the conversion of one mol of glucose to pyruvate is
thus 2 mols of ATP and 2 mols of NADH. This is the
net energy yield of glycolysis under aerobic conditions.
Under anaerobic conditions, the reduction of pyruvate to lactate
(C00186) by means of NADH oxidation is the only source of NAD+
for glyceraldehyde-3-phosphate dehydrogenase (phosphorylating)
in step 6. Depleting the cytoplasm of NAD+ effectively inhibits
glycolysis. Lactate formation is the endpoint of glycolysis in mammals.
The sustained use of skeletal muscle under stress is a typical anaerobic
reaction and the accumulation of lactate is the cause of sour muscles
after an unexpected, heavy work out.
In E.coli (and other microorganisms) the main products formed
under anaerobic conditions are in decreasing order formate,
ethanol, acetate, and succinate. While succinate is directly obtained
from phosphoenolpyruvate, formate and acetate are produced from
pyruvate. The third reaction step (shown below) is the major source
of ATP under strict anaerobic conditions and pyruvate as the only
energy source (no glucose) for this bacterium.
pyruvate
+ CoA Û acetyl-CoA + formate
acetyl-CoA
+ Pi Û acetyl-P +
CoA
acetyl-P
+ ADP Û acetate + ATP
Gluconeogenesis
(KEGG
Pathway MAP00100)
The regeneration of glucose is called gluconeogenesis and
is particularly important in liver, which is the major organ involved
in glucose synthesis from carbohydrate and non-carbohydrate
sources. It is the only organ that can regenerate glucose form lactate.
Comparing glycolysis and its reversed mode gluconeogenesis demonstrates
general rules governing metabolic pathways regarding control and
reversibility. While 7 out of 10 glycolytic steps are reversible
as they exhibit small change of free energy, three steps occur at
a considerable larger free energy change (less than -4kcal/mol)
making them irreversible. In gluconeogenesis the glycolytic enzymes
are bypassed by gluconeogenetic enzymes.
Reaction |
Glycolytic
enzyme |
Gluconeogenetic
enzyme |
Glucose
Û
glucose-6-phosphate |
Glucokinase
|
Glucose-6-phosphatase |
Fructose-6-P
Û
fructose-1,6-diP |
Phosphofructokinase
|
Fructose-1,6-biphosphatase |
Phosphoenolpyruvate
Û
pyruvate |
Pyruvate
kinase |
Pyruvate
carboxylase
Phosphoenolpyruvate carboxykinase 1
|
Comparing the energy expenditure for gluconeogenesis with the gain
from glycolysis clearly shows that it costs more to generate glucose
from internal metabolites than to break it down once glucose is
inside the cell:
1 Glucose Þ 2 Lactate
+ 2 ATP
2 Lactate + 6 ATP Þ 1 Glucose
Control of Glycolysis
and Gluconeogenesis
In mammals the balance between gluconeogenesis and glycolysis in
liver has to respond to the needs of the entire body such as mechanical
work, digestion, or thinking and thus is carefully adjusted. Here
is a list of internal and external molecules that affect the balance
between glycolysis and gluconeogenesis.
Intracellular regulators include amino
acids, the citric acid cycle intermediate citrate
and the acyl
intermediates acetyl-CoA and fatty acids. All will stimulate
gluconeogenesis through a positive feed forward control of pyruvate
carboxylase and fructose-1,6-biphosphatase, respectively, if they
are abundant. In addition, the energy charge of a cell defined by
the availability of ATP,
ADP, AMP control glycolysis and gluconeogenesis (see respiratory
control). Low energy levels (AMP, ADP) stimulate glycolysis, high
energy levels (ATP) inhibit glycolysis and activate gluconeogenesis.
Hormones are extracellular regulators which also affect
glycolysis and gluconeogenesis. They coordinate metabolic activity
among different organs. Insulin stimulates glycolysis to
lower blood glucose levels after a meal to signal energy availability
to all organs, while glucagon and epinephrine (adrenaline)
stimulate gluconeogenesis (and lipolysis in fatty tissue by hepatic
lipase) to provide energy to muscle and brain during stress.
Regulation includes enzyme modulation (phosphorylation) and enzyme
biosynthesis (gene expression).
Go to table of contents
|